Advertisement

- Next Article

Flower development before the ABC model
The abc model and flower evolution, the abc model of flower development: then and now.
- Split-screen
- Article contents
- Figures & tables
- Supplementary Data
- Peer Review
- Open the PDF for in another window
- Get Permissions
- Cite Icon Cite
- Search Site
John L. Bowman , David R. Smyth , Elliot M. Meyerowitz; The ABC model of flower development: then and now. Development 15 November 2012; 139 (22): 4095–4098. doi: https://doi.org/10.1242/dev.083972
Download citation file:
- Ris (Zotero)
- Reference Manager
In 1991, we published a paper in Development that proposed the ABC model of flower development, an early contribution to the genetic analysis of development in plants. In this, we used a series of homeotic mutants, and double and triple mutants, to establish a predictive model of organ specification in developing flowers. This model has served as the basis for much subsequent work, especially towards understanding seed plant evolution. Here, we discuss several aspects of this story, that could be a much longer one. One surprising conclusion is that materials and methods that might have led to similar work, and to the same model, were available 100 years before our experiments, belying the belief that progress in biology necessarily comes from improvements in methods, rather than in concepts.
A Development classic
The year 2012 marks 25 years since the journal Development was relaunched from its predecessor, the Journal of Embryology and Experimental Morphology ( JEEM ). In 2008, we fully digitised our Development and JEEM archives, and made them freely available online. At the same time, we took the opportunity to revisit some of the classic papers published in JEEM , in a series of commentaries (see Alfred and Smith, 2008 ). Now, to mark a quarter century of Development , we have been looking through our archives at some of the most influential papers published in Development 's pages. In this series of Spotlight articles, we have asked the authors of those articles to tell us the back-story behind their work and how the paper has influenced the development of their field. Look out for more of these Spotlight papers in the next few issues.
It is straightforward to review the state of the field of genetic approaches to flower development in the late 1980s, as we published a historical review as a background to our work – like the 1991 paper ( Bowman et al., 1991 ), this was also in Development ( Meyerowitz et al., 1989 ). We pointed out that what later would be considered to be mutants with altered flower development – in types, numbers and positions of the floral organs (sepals, petals, stamens and carpels) – were known from ancient Greece, and had been studied in great detail in the 19th century, as had normal flower development, the microscopic study of which was first extensively published by Payer in 1857 ( Payer, 1857 ). Plant genetics was of course well developed at the time, as it had been in progress since the original discoveries of Mendel in 1865. Homeotic flower variants, in which one floral organ type is replaced by another, were specifically recognized and studied in the 19th century (e.g. Masters, 1869 ) under the term ‘metamorphy’, while Bateson introduced the concept of homoeosis in 1894. A collection of homeotic mutants of snapdragon existed by the 1930s in the laboratory of Erwin Baur and co-workers ( Stubbe, 1966 ). Thus, both the materials (at least in other species than the one we used) and methods (not scanning electron microscopy, but microscopic and detailed analysis of early flower development) used in our 1991 paper were available more than 100 years earlier, and the exact materials with which cognate experiments could have been carried out existed more than 50 years earlier. The key to our model was the use of double and triple mutants. The Baur laboratory made many mutants, but none (of which we have found a record) that involved more than a single homeotic mutant.
Our paper therefore serves as a counter-example to the notion that advances in biology derive only from advances in methodology. Our 1991 work could have been carried out a century earlier, except that the conceptual framework of biology at that time (especially the notion of a regulatory gene), was such that no one had thought to do it.
We began with a set of four homeotic mutants of Arabidopsis in which fairly normal floral organs were found in floral whorls where they would not be expected in wild-type flowers. The mutants were obtained from the generosity of colleagues, particularly Maarten Koornneef, then at the University of Wageningen (The Netherlands), and had already been described in detail as single and double mutants in the first issue of The Plant Cell ( Bowman et al., 1989 ). We concluded that the genes function in overlapping fields that occupy two adjacent floral whorls, and that they ‘act in allowing cells to recognize their position in the developing flower’.
Our 1991 work could have been carried out a century earlier, except that the conceptual framework…was such that no one had thought to do it
Subsequently, additional mutant alleles were obtained and analyzed, revealing the likely null phenotypes, and triple mutants were generated ( Fig. 1 ; for further commentary, see Bowman, 2010 ). The new data were the foundation on which we built ‘A simple model’, now known as the ABC model of floral organ identity. The letters came from the three overlapping fields, named A (APETALA2 gene function, AP2), B (APETALA3 and PISTILLATA, AP3/PI) and C (AGAMOUS, AG). AP2 was proposed to function in whorl 1 to define sepals, and AG in whorl 4 to control carpel identity. The overlaps explained the combinatorial roles of AP2 and AP3/PI in whorl 2 that normally defined petals, and AP3/PI and AG in whorl 3 that specified stamens. A key new component, without which the model does not work and that distinguishes this model from others, was the proposal that genes acting in the A and C fields, AP2 and AG , were mutually antagonistic, something shown at the molecular level beginning very shortly afterwards ( Drews et al., 1991 ). It was aesthetically satisfying to be able to account for the eight floral phenotypes in terms of the model, and its attraction lay in its simplicity and predictive power. Even so, it only explained organ identity, and we listed a series of ‘unexplained complications’ associated with mutant phenotypes, including mosaic organs, secondary flowers, floral indeterminacy and missing organs.

The Arabidopsis research group at Caltech in late 1988. From left to right, Hong Ma (post-doc), Tony Bleecker (post-doc), Yi Hu (technician), John Bowman (PhD student), Usha Vijayraghavan (post-doc), Marty Yanofsky (post-doc), Elliot Meyerowitz (group leader) and David Smyth (sabbatical visitor) (absent: Sherrie Kempin, research assistant). At this time, John Bowman and David Smyth were focusing on the developmental genetics of ABC genes, and Marty Yanofsky and Hong Ma were characterizing the C function gene AGAMOUS .
We chose to send the manuscript to Development rather than to a plant-oriented journal on the encouragement of editor Keith Roberts, who seemed to share our holistic view that developmental principles are shared across kingdoms. And, color illustrations were free and there were then no page limits. The two reviewers were positive, with one saying ‘This is a stimulating paper which should have a large impact on the field. Interest rating 9.’ The other reviewer thought it ‘potentially an excellent paper’, but ‘the writing style could be improved’ and wanted it shortened by half. However, the first reviewer, and the editor, disagreed, and its 20 pages stood. We suggested a ‘fruit salad’ cover photo of the wild-type and all mutant phenotypes that was also accepted ( Fig. 2 ).

The cover of the issue of Development in which the ABC paper appeared. The photograph depicts eight different genotypes of Arabidopsis flowers. Wild-type flowers are shown with three different single floral homeotic mutants ( ap3-1, ap2-1 and ag-1 ), and the three double mutant and the triple mutant combinations of these alleles.
The ABC model was popularized in a review in Nature published later in 1991 by the senior author and Enrico Coen, whose group had been making parallel new findings of similar homeotic mutants in snapdragon ( Antirrhinum majus ) ( Coen and Meyerowitz, 1991 ). In this review, functions were distinguished from regions (or fields) by using a , b and c for functions and A, B and C for regions, although it is now customary to capitalize the functions. The importance of comparative findings in Antirrhinum was highlighted by the earlier contribution of Zsuszanna Schwarz-Sommer and colleagues, who independently generated a floral organ identity model that proposed the equivalent of B and C functions, but lacked A function and was not tested using multiple mutants ( Schwarz-Sommer et al., 1990 ).
The ABC model is still widely used as a framework for understanding floral development today ( Krizek and Fletcher, 2005 ; Causier et al., 2010 ). The impact of the Development paper is reflected in its continued high citation rate (it still gathers over 30 citations a year according to the Web of Knowledge, http://wokinfo.com ). Significant advances since 1991 include findings that: (1) all genes, except AP2 , encode MADS transcription factors (e.g. Weigel and Meyerowitz, 1994 ); (2) another A function gene, APETALA1 , exists in Arabidopsis ( Bowman et al., 1993 ; Gustafson-Brown et al., 1994 ); (3) four other MADS genes ( SEPALLATA s) are involved in establishing the floral nature of flower organs in Arabidopsis ( Pelaz et al., 2000 ) (often called E function, although using our terminology these would be meristem identity, not organ identity, genes); (4) SEP proteins likely act in multimeric combination with A, B and C function MADS proteins (the quartet model) (e.g. Melzer and Theissen 2009 ); (5) AP2 transcripts are regulated post-transcriptionally by microRNAs ( Aukerman and Sakai, 2003 ; Chen, 2004 ); and (6) AP2 is a direct negative regulator of AG expression, a very recent finding ( Dinh et al., 2012 ).
The ABC model is still widely used as a framework for understanding floral development today
Perhaps owing to its relative simplicity, and to the ubiquity of the appreciation of flowers in human society, the ABC model was rapidly introduced into university textbooks, not only those focused on developmental biology (e.g. Wolpert and Tickle, 2011 ), but also to first year general biology textbooks (e.g. Campbell et al., 1999 ; Freeman, 2008 ), cell biology texts (e.g. Alberts et al., 1994 ) and those focused on genetics (e.g. Griffiths et al., 1993 ; Sanders and Bowman, 2012 ). The ABC model is even being taught to high school students in some locales ( Fig. 3 ).

Cartoon of the ABC model drawn by Ryoko Hirano and which Hiroyuki Hirano used to teach summer school for secondary school students in Japan. Note the colorful inclusion of the critical A-C mutual inhibition. Image courtesy of Hiroyuki and Ryoko Hirano (University of Tokyo).
Given the conservation of floral organ position across angiosperms, it was a natural question as ask whether the ABC model could be applied to all flowering plants – and not only to Arabidopsis and snapdragon, where it was developed. Its integration with new findings that were, at that time, defining the course of angiosperm evolution was catalyzed by two Keystone Symposia on evolution and plant development (early evo-devo) held at Taos (New Mexico) in 1993 and 1997. Two early tests of its general applicability were observations that showed the occurrence of B-class mutants in monocots ( Ambrose et al., 2000 ; van Tunen et al., 1993 ). Such observations formed the foundation for continuing studies into the diversity of flower architecture, from orchids where the complex perianth is patterned by differential expression of multiple B-class gene paralogs ( Mondragon-Palomino and Theissen, 2011 ), to the ‘inside-out’ flowers of Lacandonia schismatica , where central stamens are surrounded by carpels ( Alvarez-Buylla et al., 2010 ).
The ABC model has also illuminated the evolutionary appearance of flowers, a puzzle that has occupied botanists since Charles Darwin. Both B- and C-class orthologs are found in gymnosperms, with C-class genes expressed in both male and female reproductive structures and B-class expression in male reproductive tissues ( Mouradov et al., 1999 ; Shindo et al., 1999 ; Sundstrom et al., 1999 ; Tandre et al., 1998 ). Thus, the specification of stamens and carpels seems to have its origin in the common ancestor of seed plants, and the mystery of flower evolution lies in ‘tinkering’ ( Jacob, 1977 ) with pre-existing genetic machinery. None of the ABC-type MADS box genes appears to exist outside angiosperms and gymnosperms, suggesting that their origin lies in extensive gene duplications of an ancestral MADS box gene in the lineage leading to seed plants ( Floyd and Bowman, 2007 ).
In summary, althought the relatively simple ABC model we proposed in 1991 has grown in complexity over the past 21 years – particularly with increasing knowledge of its molecular mechanism – it still provides a basis for our understanding of flower development and morphology in model systems, and across evolution.
Email alerts
Pathway to independence programme: our 2024 pi fellows.

Following a successful pilot year in 2023 with a fantastic set of postdocs , we are delighted to announce our second cohort of Pathway to Independence (PI) fellows, who we will be supporting with training, mentoring and networking opportunities over the coming years.
Development presents… Outstanding Paper Prize finalists

On 21 May, we celebrate the winners of Development’s 2023 Outstanding Paper Prize in a special edition of our Development presents webinar series, hearing from the authors of two papers describing the roles of the Frizzled receptors in development, evolution and disease. Register for the webinar .
Propose a new Workshop for 2026

We are now accepting proposals for our 2026 Workshops programme. We aim to be responsive to the community and open to novel initiatives, so if you have a new idea for a biological workshop that you feel would work well, please apply . Applications deadline: 19 July 2024.
SciCommConnect: Science communication, community connections

Sign up now for SciCommConnect, a free half-day event hosted by The Company of Biologists’ community sites – The Node, preLights, and FocalPlane – on 10 June 2024. The programme will feature insightful discussions, relevant talks and an exciting Three Minute Research Talk Competition.
Biologists @ 100 - join us in Liverpool in March 2025

We are excited to invite you to a unique scientific conference, celebrating the 100-year anniversary of The Company of Biologists, and bringing together our different communities. The conference will incorporate the Spring Meetings of the BSCB and the BSDB, the JEB Symposium Sensory Perception in a Changing World and a DMM programme on antimicrobial resistance. Find out more and register your interest to join us in March 2025 in Liverpool, UK.
Social media

Other journals from The Company of Biologists
- Journal of Cell Science
- Journal of Experimental Biology
- Disease Models & Mechanisms
- Biology Open
- About Development
- Editors and Board
- Aims and scope
- Submit a manuscript
- Manuscript preparation
- Journal policies
- Rights and permissions
- Sign up for alerts
Affiliations
- Development
- Journal Meetings
- Library hub
- Company news
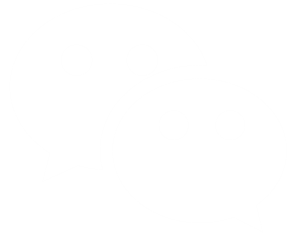
- Privacy policy
- Terms & conditions
- Copyright policy
- © 2024 The Company of Biologists. All rights reserved.
- Registered Charity 277992 | Registered in England and Wales | Company Limited by Guarantee No 514735 Registered office: Bidder Building, Station Road, Histon, Cambridge CB24 9LF, UK
This Feature Is Available To Subscribers Only
Sign In or Create an Account
Thank you for visiting nature.com. You are using a browser version with limited support for CSS. To obtain the best experience, we recommend you use a more up to date browser (or turn off compatibility mode in Internet Explorer). In the meantime, to ensure continued support, we are displaying the site without styles and JavaScript.
- View all journals
- Explore content
- About the journal
- Publish with us
- Sign up for alerts
- Review Article
- Published: 01 September 2005
Molecular mechanisms of flower development: an armchair guide
- Beth A. Krizek 1 &
- Jennifer C. Fletcher 2 , 3
Nature Reviews Genetics volume 6 , pages 688–698 ( 2005 ) Cite this article
9149 Accesses
438 Citations
4 Altmetric
Metrics details
Floral-meristem identity genes promote flower development by repressing genes that promote an inflorescence fate and activate floral-organ identity genes.
The updated ABCE model of floral-organ identity states that SEPALLATA (E class) genes function with different combinations of the A, B and C class floral-organ identity genes to specify sepal, petal, stamen and carpel identity.
Most of the ABCE class floral-organ identity genes encode MADS domain proteins, which form multimeric transcriptional regulatory complexes.
Regulation of floral-organ identity gene expression is complex and occurs at multiple levels including post-translational regulation by microRNAs.
New approaches have begun to reveal downstream targets of floral-organ identity genes, but the complex regulatory cascades that control floral organogenesis are not well understood.
Molecular genetic studies are starting to reveal the mechanisms that are behind other aspects of flower development, such as the regulation of organ size and the generation of floral symmetry.
Regulation of floral-organ identity seems to occur through similar mechanisms in eudicots and monocots.
An afternoon stroll through an English garden reveals the breathtaking beauty and enormous diversity of flowering plants. The extreme variation of flower morphologies, combined with the relative simplicity of floral structures and the wealth of floral mutants available, has made the flower an excellent model for studying developmental cell-fate specification, morphogenesis and tissue patterning. Recent molecular genetic studies have begun to reveal the transcriptional regulatory cascades that control early patterning events during flower formation, the dynamics of the gene-regulatory interactions, and the complex combinatorial mechanisms that create a distinct final floral architecture and form.
This is a preview of subscription content, access via your institution
Access options
Subscribe to this journal
Receive 12 print issues and online access
176,64 € per year
only 14,72 € per issue
Buy this article
- Purchase on Springer Link
- Instant access to full article PDF
Prices may be subject to local taxes which are calculated during checkout

Similar content being viewed by others

Developmental mechanisms involved in the diversification of flowers

TERMINAL FLOWER 1-FD complex target genes and competition with FLOWERING LOCUS T

LEAFY is a pioneer transcription factor and licenses cell reprogramming to floral fate
Ng, M. & Yanofsky, M. F. Three ways to learn the ABCs. Curr. Opin. Plant Biol. 3 , 47–52 (2000).
Article CAS PubMed Google Scholar
Lohmann, J. U. & Weigel, D. Building beauty: the genetic control of floral patterning. Dev. Cell 2 , 135–142 (2002). This review presents an in-depth examination of the floral-organ identity genes and their regulation .
Theissen, G. Development of floral organ identity: stories from the MADS house. Curr. Opin. Plant Biol. 4 , 75–85 (2001).
Steeves, T. A. & Sussex, I. M. Patterns in Plant Development (Cambridge Univ. Press, New York, 1989).
Book Google Scholar
Jurgens, G. Apical–basal pattern formation in Arabidopsis embryogenesis. EMBO J. 20 , 3609–3616 (2001).
Article CAS PubMed PubMed Central Google Scholar
Fletcher, J. C. Shoot and floral meristem maintenance in Arabidopsis . Annu. Rev. Plant Biol. 53 , 45–66 (2002).
Simpson, G. G. & Dean, C. Arabidopsis , the Rosetta stone of flowering time. Science 296 , 285–289 (2002).
Coen, E. S. et al. floricaula : a homeotic gene required for flower development in Antirrhinum majus . Cell 63 , 1311–1322 (1990).
Mandel, M. A. & Yanofsky, M. F. A gene triggering flower formation in Arabidopsis . Nature 377 , 522–524 (1995).
Weigel, D., Alvarez, J., Smyth, D. R., Yanofsky, M. F. & Meyerowitz, E. M. LEAFY controls floral meristem identity in Arabidopsis . Cell 69 , 843–859 (1992).
Weigel, D. & Nilsson, O. A developmental switch sufficient for flower initiation in diverse plants. Nature 377 , 495–500 (1995).
Huijser, P. et al. Bracteomania, an inflorescence anomaly, is caused by the loss of function of the MADS-box gene squamosa in Antirrhinum majus . EMBO J. 11 , 1239–1249 (1992).
Parcy, F., Nilsson, O., Busch, M. A., Lee, I. & Weigel, D. A genetic framework for floral patterning. Nature 395 , 561–566 (1998).
Riechmann, J. L., Krizek, B. A. & Meyerowitz, E. M. Dimerization specificity of Arabidopsis MADS domain homeotic proteins APETALA1, APETALA3, PISTILLATA, and AGAMOUS. Proc. Natl Acad. Sci. USA 93 , 4793–4798 (1996).
Liljegren, S. J., Gustafson-Brown, C., Pinyopich, A., Ditta, G. S. & Yanofsky, M. F. Interactions among APETALA1 , LEAFY and TERMINAL FLOWER1 specify meristem fate. Plant Cell 11 , 1007–1018 (1999).
Wagner, D., Sablowski, R. W. M. & Meyerowitz, E. M. Transcriptional activation of APETALA1 by LEAFY. Science 285 , 582–584 (1999).
William, D. A. et al. Genomic identification of direct targets of LEAFY. Proc. Natl Acad. Sci. USA 101 , 1775–1780 (2004). This paper describes the use of microarray analysis to identify putative LEAFY primary target genes followed by chromatin immunoprecipitation to confirm the binding of the LEAFY protein to the target-gene promoters .
Ratcliffe, O. J., Bradley, D. J. & Coen, E. S. Separation of shoot and floral identity in Arabidopsis . Development 126 , 1109–1120 (1999).
Yu, H., Ito, T., Wellmer, F. & Meyerowitz, E. M. Repression of AGAMOUS-LIKE 24 is a crucial step in promoting flower development. Nature Genet. 36 , 157–161 (2004).
Hanzawa, Y., Money, T. & Bradley, D. A single amino acid converts a repressor to an activator of flowering. Proc. Natl Acad. Sci. USA 102 , 7748–7753 (2005).
Schmid, M. et al. Dissection of floral induction pathways using global expression analysis. Development 130 , 6001–6012 (2003).
Wagner, D. et al. Floral induction in tissue culture: a system for the analysis of LEAFY-dependent gene regulation. Plant J. 39 , 273–282 (2004).
Coen, E. S. & Meyerowitz, E. M. The war of the whorls — genetic interactions controlling flower development. Nature 353 , 31–37 (1991).
Keck, E., McSteen, P., Carpenter, R. & Coen, E. Separation of genetic functions controlling organ identity in flowers. EMBO J. 22 , 1058–1066 (2003).
Kanno, A., Saeki, H., Kameya, T., Saedler, H. & Theissen, G. Heterotropic expression of class B floral homeotic genes supports a modified ABC model for tulip (Tulipa gesneriana) . Plant Mol. Biol. 52 , 831–841 (2003).
Angenent, G. C., Busscher, M., Franken, J., Mol, J. N. M. & van Tunen, A. J. Differential expression of two MADS box genes in wild-type and mutant Petunia flowers. Plant Cell 4 , 983–993 (1992).
CAS PubMed PubMed Central Google Scholar
Webster, M. A. & Gilmartin, P. M. A comparison of early floral ontogeny in wild-type and floral homeotic mutant phenotypes of Primula. Planta 216 , 903–917 (2003).
Nagasawa, N. et al. SUPERWOMAN and DROOPING LEAF genes control floral organ identity in rice. Development 130 , 705–718 (2003).
Whipple, C. J. et al. Conservation of B-class floral homeotic gene function between maize and Arabidopsis . Development 131 , 6083–6091 (2004).
Ditta, G., Pinyopich, A., Robles, P., Pelaz, S. & Yanofsky, M. F. The SEP4 gene of Arabidopsis thaliana functions in floral organ and meristem identity. Curr. Biol. 14 , 1935–1940 (2004). This study identifies a fourth SEP gene, which has an important role for specification of floral-meristem identity, and showed that SEP genes are required for specification of all floral-organ identities .
Bowman, J. L., Smyth, D. R. & Meyerowitz, E. M. Genetic interactions among floral homeotic genes of Arabidopsis . Development 112 , 1–20 (1991).
Vandenbussche, M. et al. Toward the analysis of the petunia MADS box gene family by reverse and forward transposon mutagenesis approaches: B, C and D floral organ identity functions require SEPALLATA -like MADS box genes in petunia. Plant Cell 15 , 2686–2693 (2003).
Article CAS Google Scholar
Ferrario, S., Immink, R. G. H., Shchennikova, A., Busscher-Lange, J. & Angenent, G. C. The MADS box gene FBP2 is required for SEPALLATA function in petunia. Plant Cell 15 , 914–925 (2003).
Colombo, L. et al. The petunia MADS box gene FBP11 determines ovule identity. Plant Cell 7 , 1859–1868 (1995).
Pinyopich, A. et al. Assessing the redundancy of MADS-box genes during carpel and ovule development. Nature 424 , 85–88 (2003).
Favaro, R. et al. MADS-box protein complexes control carpel and ovule development in Arabidopsis . Plant Cell 15 , 2603–2611 (2003).
Parenicova, L. et al. Molecular and phylogenetic analyses of the complete MADS-box transcription factor family in Arabidopsis : new openings to the MADS world. Plant Cell 15 , 1538–1551 (2003).
Honma, T. & Goto, K. Complexes of MADS-box proteins are sufficient to convert leaves into floral organs. Nature 409 , 525–529 (2001). This paper describes the association of MADS domain proteins into multimeric complexes (A + B + E and B + C + E). Ectopic expression of the class A, B, C and E floral-organ identity genes was shown to be sufficient to convert leaves into reproductive organs .
Pelaz, S., Tapia-Lopez, R., Alvarez-Buylla, E. R. & Yanofsky, M. F. Conversion of leaves into petals in Arabidopsis . Curr. Biol. 11 , 182–184 (2001).
Honma, T. & Goto, K. The Arabidopsis floral homeotic gene PISTILLATA is regulated by discrete cis -elements responsive to induction and maintenance signals. Development 127 , 2021–2030 (2000).
Egea-Cortines, M., Saedler, H. & Sommer, H. Ternary complex formation between the MADS-box proteins SQUAMOSA, DEFICIENS and GLOBOSA is involved in the control of floral architecture in Antirrhinum majus . EMBO J. 18 , 5370–5379 (1999).
Pelaz, S., Ditta, G. S., Baumann, E., Wisman, E. & Yanofsky, M. F. B and C floral organ identity functions require SEPALLATA MADS-box genes. Nature 405 , 200–203 (2000). This paper defines a new organ-identity function. The SEPALLATA genes ( SEP1 , SEP2 , SEP3 ) function with the class A, B and C floral-organ identity genes to specify petal, stamen and carpel identity .
Immink, R. G. H. et al. Analysis of the petunia MADS box transcription factor family. Mol. Genet. Genomics 268 , 598–606 (2003).
Fan, H. -Y., Hu, Y., Tudor, M. & Ma, H. Specific interactions between the K domains of AG and the AGLs, members of the MADS domain family of DNA binding proteins. Plant J. 11 , 999–1010 (1997).
Article Google Scholar
Pelaz, S., Gustafson-Brown, C., Kohalmi, S. E., Crosby, W. L. & Yanofsky, M. F. APETALA1 and SEPALLATA3 interact to promote flower development. Plant J. 26 , 385–394 (2001).
de Folter, S. et al. Comprehensive interaction map of the Arabidopsis MADS box transcription factors. Plant Cell 17 , 1424–1433 (2005). A comprehensive analysis of interactions between MADS domain proteins. Because of the observed interactions between some MADS domain proteins that specify floral-organ fate with those that regulate floral induction, the authors propose a model in which such mixed complexes function in negative-feedback loops to control switches in meristem identity .
Ng, M. & Yanofsky, M. F. Activation of the Arabidopsis B class homeotic genes by APETALA1 . Plant Cell 13 , 739–753 (2001).
Lenhard, M., Bohnert, A., Jurgens, G. & Laux, T. Termination of stem cell maintenance in Arabidopsis floral meristems by interactions between WUSCHEL and AGAMOUS . Cell 105 , 805–814 (2001).
Lohmann, J. U. et al. A molecular link between stem cell regulation and floral patterning in Arabidopsis . Cell 105 , 793–803 (2001). This paper, together with reference 48, shows that termination of meristem identity in the centre of a flower is due to downregulation of the stem cell specifier WUSCHEL by the floral-organ identity gene AGAMOUS .
Alvarez-Venegas, R. et al. ATX-1, an Arabidopsis homolog of trithorax, activates flower homeotic genes. Curr. Biol. 13 , 627–637 (2003).
Yu, H. et al. Floral homeotic genes are targets of gibberellin signaling in flower development. Proc. Natl Acad. Sci. USA 101 , 7827–7832 (2004).
Gustafson-Brown, C., Savidge, B. & Yanofsky, M. F. Regulation of the Arabidopsis floral homeotic gene APETALA1 . Cell 76 , 131–143 (1994).
Drews, G. N., Bowman, J. L. & Meyerowitz, E. M. Negative regulation of the Arabidopsis homeotic gene AGAMOUS by the APETALA2 product. Cell 65 , 991–1001 (1991).
Aukerman, M. J. & Sakai, H. Regulation of flowering time and floral organ identity by a microRNA and its APETALA2 -like target genes. Plant Cell 15 , 2730–2741 (2003).
Chen, X. A microRNA as a translational repressor of APETALA2 in Arabidopsis flower development. Science 303 , 2022–2025 (2004). This paper, together with reference 54, describes the role of an miRNA in regulating floral-organ identity gene expression .
Schwab, R. et al. Specific effects of microRNAs on the plant transcriptome. Dev. Cell 8 , 517–527 (2005).
Sridhar, V. V., Surendrarao, A., Gonzalez, D., Conlan, R. S. & Liu, Z. Transcriptional repression of target genes by LEUNIG and SEUSS, two interacting regulatory proteins for Arabidopsis flower development. Proc. Natl Acad. Sci. USA 101 , 11494–11499 (2004).
Navarro, C. et al. Molecular and genetic interactions between STYLOSA and GRAMINIFOLIA in the control of Antirrhinum vegetative and reproductive development. Development 131 , 3649–3659 (2004).
Byzova, M. V. et al. Arabidopsis STERILE APETALA , a multifunctional gene regulating inflorescence, flower and ovule development. Genes Dev. 13 , 1002–1014 (1999).
Krizek, B. A., Prost, V. & Macias, A. AINTEGUMENTA promotes petal identity and acts as a negative regulator of AGAMOUS . Plant Cell 12 , 1357–1366 (2000).
Bao, X., Franks, R. G., Levin, J. Z. & Liu, Z. Repression of AGAMOUS by BELLRINGER in floral and inflorescence meristems. Plant Cell 16 , 1478–1489 (2004).
Bowman, J. L. et al. SUPERMAN , a regulator of floral homeotic genes in Arabidopsis . Development 114 , 599–615 (1992).
Schultz, E. A., Pickett, F. B. & Haughn, G. W. The FLO10 gene-product regulates the expression domain of homeotic genes AP3 and PI in Arabidopsis flowers. Plant Cell 3 , 1221–1237 (1991).
Sakai, H., Medrano, L. J. & Meyerowitz, E. M. Role of SUPERMAN in maintaining Arabidopsis floral whorl boundaries. Nature 378 , 199–203 (1995).
Chen, X. & Meyerowitz, E. M. HUA1 and HUA2 are two members of the floral homeotic AGAMOUS pathway. Mol. Cell 3 , 349–360 (1999).
Cheng, Y. & Chen, X. Posttranscriptional control of plant development. Curr. Opin. Plant Biol. 7 , 20–25 (2004).
Cheng, Y., Kato, N., Wang, W., Li, J. & Chen, X. Two RNA binding proteins, HEN4 and HUA1, act in the processing of AGAMOUS pre-mRNA in Arabidopsis thaliana . Dev. Cell 4 , 53–66 (2003).
Park, M. Y., Wu, G., Gonzalez-Sulser, A., Vaucheret, H. & Poethig, R. S. Nuclear processing and export of microRNAs in Arabidopsis . Proc. Natl Acad. Sci. USA 102 , 3691–3696 (2005).
Yu, B. et al. Methylation as a crucial step in plant microRNA biogenesis. Science 307 , 932–935 (2005).
Schoof, H. et al. The stem cell population of Arabidopsis shoot meristems is maintained by a regulatory loop between the CLAVATA and WUSCHEL genes. Cell 100 , 635–644 (2000).
Carles, C. C., Choffnes-Inada, D., Reville, K., Lertpiriyapong, K. & Fletcher, J. C. ULTRAPETALA1 encodes a putative SAND domain transcription factor that controls shoot and floral meristem activity in Arabidopsis . Development 132 , 897–911 (2005).
Fletcher, J. C. The ULTRAPETALA gene controls shoot and floral meristem size in Arabidopsis . Development 128 , 1323–1333 (2001).
Carles, C. C., Lertpiriyapong, K., Reville, K. & Fletcher, J. C. The ULTRAPETALA1 gene functions early in Arabidopsis development to restrict shoot apical meristem activity, and acts through WUSCHEL to regulate floral meristem determinacy. Genetics 167 , 1893–1903 (2004).
Goodrich, J. et al. A Polycomb-group gene regulates homeotic gene expression in Arabidopsis . Nature 386 , 44–51 (1997).
Hennig, L., Taranto, P., Walser, M., Schonrock, N. & Gruissem, W. Arabidopsis MSI1 is required for epigenetic maintenance of reproductive development. Development 130 , 2555–2565 (2003).
Serrano-Cartegena, J. et al. Genetic analysis of incurvata mutants reveals three independent genetic operations at work in Arabidopsis leaf morphogenesis. Genetics 156 , 1363–1377 (2000).
Chitvivattana, Y. et al. Interaction of Polycomb-group proteins controlling flowering in Arabidopsis . Development 131 , 5263–5276 (2004).
Lund, A. H. & van Lohuizen, M. Polycomb complexes and silencing mechanisms. Curr. Opin. Cell Biol. 16 , 239–246 (2004).
Sablowski, R. W. M. & Meyerowitz, E. M. A homologue of NO APICAL MERISTEM is an immediate target of the floral homeotic genes APETALA3/PISTILLATA . Cell 92 , 93–103 (1998).
Sakai, H., Krizek, B. A., Jacobsen, S. E. & Meyerowitz, E. M. Regulation of SUP expression identifies multiple regulators involved in Arabidopsis floral meristem development. Plant Cell 12 , 1607–1618 (2000).
Zik, M. & Irish, V. Global identification of target genes regulated by APETALA3 and PISTILLATA floral homeotic gene action. Plant Cell 15 , 207–222 (2003).
Ito, T. et al. The homeotic protein AGAMOUS controls microsporogenesis by regulation of SPOROCYTELESS . Nature 430 , 356–360 (2004).
Gomez-Mena, C., de Folter, S., Costa, M. M., Angenent, G. C. & Sablowski, R. Transcriptional program controlled by the floral homeotic gene AGAMOUS during early organogenesis. Development 132 , 429–438 (2005).
Cheng, H. et al. Gibberellin regulates Arabidopsis floral development via suppression of DELLA protein function. Development 131 , 1055–1064 (2004).
Wellmer, F., Riechmann, J. L., Alves-Ferreira, M. & Meyerowitz, E. M. Genome-wide analysis of spatial gene expression in Arabidopsis flowers. Plant Cell 16 , 1314–1326 (2004). This paper describes a microarray approach that uses floral-homeotic mutants to identify genes that are expressed in an organ-specific manner .
Durfee, T. et al. The F-box-containing protein UFO and AGAMOUS participate in antagonistic pathways governing early petal development in Arabidopsis . Proc. Natl Acad. Sci. USA 100 , 8571–8576 (2003).
Griffith, M. E., da Silva Conceicao, A. & Smyth, D. R. PETAL LOSS gene regulates initiation and orientation of second whorl organs in the Arabidopsis flower. Development 126 , 5635–5644 (1999).
Takeda, S., Matsumoto, N. & Okada, K. RABBIT EARS , encoding a SUPERMAN-like zinc finger protein, regulates petal development in Arabidopsis thaliana . Development 131 , 425–434 (2003).
Article PubMed CAS Google Scholar
Xing, S., Rosso, M. G. & Zachgo, S. ROXY1 , a member of the plant glutaredoxin family, is required for petal development in Arabidopsis thaliana . Development 132 , 1555–1565 (2005).
Brewer, P. B. et al. PETAL LOSS , a trihelix transcription factor gene, regulates perianth architecture in the Arabidopsis flower. Development 131 , 4035–4045 (2004).
Aida, M., Ishida, T., Fukaki, H., Fujisawa, H. & Tasaka, M. Genes involved in organ separation in Arabidopsis : an analysis of the cup-shaped cotyledon mutant. Plant Cell 9 , 841–857 (1997).
Souer, E., van Houwelingen, A., Kloos, D., Mol, J. & Koes, R. The NO APICAL MERISTEM gene of Petunia is required for pattern formation in embryos and flowers and is expressed at meristem and primordia boundaries. Cell 85 , 159–170 (1996).
Takada, S., Hibara, K., Ishida, T. & Tasaka, M. The CUP-SHAPED COTYLEDON1 gene of Arabidopsis regulates shoot apical meristem formation. Development 128 , 1127–1135 (2001).
Weir, I. et al. CUPULIFORMIS establishes lateral organ boundaries in Antirrhinum . Development 131 , 915–922 (2004).
Cubas, P., Lauter, N., Doebley, J. & Coen, E. The TCP domain: a motif found in proteins regulating plant growth and development. Plant J. 18 , 215–222 (1999).
Rhoades, M. W. et al. Prediction of plant microRNA targets. Cell 110 , 513–520 (2002).
Baker, C. C., Sieber, P., Wellmer, F. & Meyerowitz, E. M. The early extra petals1 mutant uncovers a role for microRNA miR164c in regulating petal number in Arabidopsis . Curr. Biol. 15 , 303–315 (2005).
Laufs, P., Peaucelle, A., Morin, H. & Traas, J. MicroRNA regulation of the CUC genes is required for boundary size control in Arabidopsis meristems. Development 131 , 4311–4322 (2004).
Mallory, A. C., Dugas, D. V., Bartel, D. P. & Bartel, B. MicroRNA regulation of NAC-domain targets is required for proper formation and separation of adjacent embryonic, vegetative and floral organs. Curr. Biol. 14 , 1035–1046 (2004).
Soltis, D. E. et al. Missing links: the genetic architecture of flower and floral diversification. Trends Plant Sci. 7 , 22–31 (2002).
van Tunen, A. J., Eikelboom, W. & Angenent, G. Floral organogenesis in Tulipa. Flow. News Lett. 16 , 33–37 (1993).
Google Scholar
Ochiai, T. et al. The differentiation of sepal and petal morphologies in Commelinaceae. Gene 343 , 253–262 (2004).
Ambrose, B. A. et al. Molecular and genetic analyses of the silky1 gene reveal conservation in floral organ specification between eudicots and monocots. Mol. Cell 5 , 569–579 (2000).
Mena, M. et al. Diversification of C-function activity in maize flower development. Science 274 , 1537–1540 (1996).
Kang, H. -G., Jeon, J. -S., Lee, S. & An, G. Identification of class B and class C floral organ identity genes from rice plants. Plant Mol. Biol. 38 , 1021–1029 (1998).
Yamaguchi, T. et al. The YABBY gene DROOPING LEAF regulates carpel specification and midrib development in Oryza sativa . Plant Cell 16 , 500–509 (2004). This paper identifies a novel role for a YABBY gene in carpel identity specification in rice .
Tsukaya, H. Organ shape and size: a lesson from studies of leaf morphogenesis. Curr. Opin. Plant Biol. 6 , 57–62 (2003).
Article PubMed Google Scholar
Hu, Y., Xie, A. & Chua, N. -H. The Arabidopsis auxin-inducible gene ARGOS controls lateral organ size. Plant Cell 15 , 1951–1961 (2003).
Elliot, R. C. et al. AINTEGUMENTA , an APETALA2 -like gene of Arabidopsis with pleiotropic roles in ovule development and floral organ growth. Plant Cell 8 , 155–168 (1996).
Klucher, K. M., Chow, H., Reiser, L. & Fischer, R. L. The AINTEGUMENTA gene of Arabidopsis required for ovule and female gametophyte development is related to the floral homeotic gene APETALA2 . Plant Cell 8 , 137–153 (1996).
Krizek, B. A. Ectopic expression of AINTEGUMENTA in Arabidopsis plants results in increased growth of floral organs. Dev. Genet. 25 , 224–236 (1999).
Mizukami, Y. & Fischer, R. L. Plant organ size control: AINTEGUMENTA regulates growth and cell numbers during organogenesis. Proc. Natl Acad. Sci. USA 97 , 942–947 (2000).
Waites, R. & Hudson, A. phantastica: a gene required for dorsoventrality of leaves in Antirrhinum majus . Development 121 , 2143–2154 (1995).
Golz, J. F., Roccaro, M., Kuzoff, R. & Hudson, A. GRAMINIFOLIA promotes growth and polarity of Antirrhinum leaves. Development 131 , 3661–3670 (2004).
Siegfried, K. R. et al. Members of the YABBY gene family specify abaxial cell fate in Arabidopsis . Development 126 , 4117–4128 (1999).
Crawford, B. C. W., Nath, U., Carpenter, R. & Coen, E. CINCINNATA controls both cell differentiation and growth in petal lobes and leaves of Antirrhinum . Plant Physiol. 135 , 244–253 (2004).
Juenger, T., Perez-Perez, J. M., Bernal, S. & Micol, J. L. Quantitative trait loci mapping of floral and leaf morphology traits in Arabidopsis thaliana : evidence for modular genetic architecture. Evol. Dev. 7 , 259–271 (2005).
Linnaeus, C. De Peloria (Diss. Ac. Amoenitates Academicae III, Uppsala, 1749).
Galego, L. & Almeida, J. Role of DIVARICATA in the control of dorsoventral symmetry in Antirrhinum flowers. Genes Dev. 16 , 880–891 (2002).
Perez-Rodriguez, M., Jaffe, F. W., Butelli, E., Glover, B. J. & Martin, C. Development of three different cell types is associated with the activity of a specific MYB transcription factor in the ventral petal of Antirrhinum majus flowers. Development 132 , 359–370 (2005).
Luo, D., Carpenter, R., Vincent, C., Copsey, L. & Coen, E. Origin of floral asymmetry in Antirrhinum . Nature 383 , 794–799 (1996).
Luo, D. et al. Control of organ asymmetry in flowers of Antirrhinum . Cell 99 , 367–376 (1999).
Corley, S. B., Carpenter, R., Copsey, L. & Coen, E. Floral asymmetry involves an interplay between TCP and MYB transcription factors in Antirrhinum . Proc. Natl Acad. Sci. USA 102 , 5068–5073 (2005).
Cubas, P., Vincent, C. & Coen, E. An epigenetic mutation responsible for natural variation in floral symmetry. Nature 401 , 157–161 (1999).
Jofuku, K. D., den Boer, B. G. W., van Montagu, M. & Okamuro, J. K. Control of Arabidopsis flower and seed development by the homeotic gene APETALA2 . Plant Cell 6 , 1211–1225 (1994).
Mandel, M. A., Gustafson-Brown, C., Savidge, B. & Yanofsky, M. F. Molecular characterization of the Arabidopsis floral homeotic gene APETALA1 . Nature 360 , 273–277 (1992).
Goto, K. & Meyerowitz, E. M. Function and regulation of the Arabidopsis floral homeotic gene PISTILLATA . Genes Dev. 8 , 1548–1560 (1994).
Jack, T., Brockman, L. L. & Meyerowitz, E. M. The homeotic gene APETALA3 of Arabidopsis thaliana encodes a MADS box and is expressed in petals and stamens. Cell 68 , 683–697 (1992).
Weigel, D. & Meyerowitz, E. M. Activation of floral homeotic genes in Arabidopsis . Science 261 , 1723–1726 (1993).
Yanofsky, M. F. et al. The protein encoded by the Arabidopsis homeotic gene agamous resembles transcription factors. Nature 346 , 35–39 (1990).
Flanagan, C. A. & Ma, H. Spatially and temporally regulated expression of the MADS box gene AGL2 in wild-type and mutant Arabidopsis flowers. Plant Mol. Biol. 26 , 581–595 (1994).
Savidge, B., Rounsley, S. D. & Yanofsky, M. F. Temporal relationship between the transcription of two Arabidopsis MADS box genes and the floral organ identity genes. Plant Cell 7 , 721–33 (1995).
Mandel, M. A. & Yanofsky, M. F. The Arabidopsis AGL9 MADS box gene is expressed in young flower primordia. Sex. Plant Reprod. 11 , 22–28 (1998).
Download references
Acknowledgements
The authors thank E. Coen, G. Ditta, M. Yanofsky, G. Angenent and P. McSteen for supplying photographs, and Y. Eshed for communicating results prior to publication. We acknowledge support for our work from the United States Department of Agriculture, the National Science Foundation, and the United States Department of Energy.
Author information
Authors and affiliations.
Department of Biological Sciences, University of South Carolina, 700 Sumter Street, Columbia, 29208, South Carolina, USA
Beth A. Krizek
USDA-ARS Plant Gene Expression Center, 800 Buchanan Street, Albany, 94710, California
Jennifer C. Fletcher
Department of Plant and Microbial Biology, University of California Berkeley, California, 94720, USA
You can also search for this author in PubMed Google Scholar
Corresponding author
Correspondence to Jennifer C. Fletcher .
Ethics declarations
Competing interests.
The authors declare no competing financial interests.
Related links
Further information.
Arabidopsis 2010–Establishing regulatory networks in Arabidopsis
Beth Krizek's web site
Elliot Meyerowitz's laboratory
Enrico Coen's laboratory
Floral Genome Project
Maize Genetics and Genomics Database
Martin Yanofsky's laboratory
Max Planck Institute for Plant Breeding Research
The Arabidopsis Information Resource
The Fletcher Laboratory
A method to determine the in vivo binding of a protein to a DNA sequence.
The growing shoot tip during the reproductive, flower-producing phase of development.
A concentric ring of floral organs.
The outermost sterile floral organs that include sepals, petals and tepals.
The organ of a flower perianth in which first and second-whorl organs have the same form.
The organs within the carpels that contain the embryo sac and develop into seeds.
A group of cells that is initiated by the inflorescence meristem that generates the organs of a flower.
A structure undergoing growth without a defined end.
A shoot that contains more than one flower.
An event in which an organ of one type assumes the identity of another type within a meristic series.
A plant that forms two seed leaves during embryogenesis and has three or more pores in its pollen.
A plant that forms a single seed leaf during embryogenesis.
This model proposes that tetrameric complexes of MADS proteins determine floral-organ identity in each whorl.
A gene activity that defines the boundaries of a region within a flower.
A molecular method to identify sequences that are enriched in one genotype or set of conditions compared with another.
Rights and permissions
Reprints and permissions
About this article
Cite this article.
Krizek, B., Fletcher, J. Molecular mechanisms of flower development: an armchair guide. Nat Rev Genet 6 , 688–698 (2005). https://doi.org/10.1038/nrg1675
Download citation
Issue Date : 01 September 2005
DOI : https://doi.org/10.1038/nrg1675
Share this article
Anyone you share the following link with will be able to read this content:
Sorry, a shareable link is not currently available for this article.
Provided by the Springer Nature SharedIt content-sharing initiative
This article is cited by
Seasonal variation of two floral patterns in clematis ‘vyvyan pennell’ and its underlying mechanism.
BMC Plant Biology (2024)
HB31 and HB21 regulate floral architecture through miRNA396/GRF modules in Arabidopsis
- Young Koung Lee
- Andrew Olson
- Doreen Ware
Plant Biotechnology Reports (2024)
Seagrass genomes reveal ancient polyploidy and adaptations to the marine environment
- Steffen Vanneste
- Yves Van de Peer
Nature Plants (2024)
A rapid and sensitive, multiplex, whole mount RNA fluorescence in situ hybridization and immunohistochemistry protocol
- Bruno Guillotin
- Doris Wagner
Plant Methods (2023)
De novo transcriptome assembly reveals characteristics of flower sex determination of Excoecaria agallocha
Annals of Forest Science (2022)
Quick links
- Explore articles by subject
- Guide to authors
- Editorial policies
Sign up for the Nature Briefing newsletter — what matters in science, free to your inbox daily.

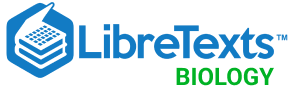
- school Campus Bookshelves
- menu_book Bookshelves
- perm_media Learning Objects
- login Login
- how_to_reg Request Instructor Account
- hub Instructor Commons
Margin Size
- Download Page (PDF)
- Download Full Book (PDF)
- Periodic Table
- Physics Constants
- Scientific Calculator
- Reference & Cite
- Tools expand_more
- Readability
selected template will load here
This action is not available.
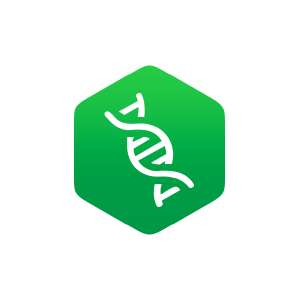
30.12: Plant Development - Genetic Control of Flowers
- Last updated
- Save as PDF
- Page ID 13758
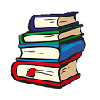
\( \newcommand{\vecs}[1]{\overset { \scriptstyle \rightharpoonup} {\mathbf{#1}} } \)
\( \newcommand{\vecd}[1]{\overset{-\!-\!\rightharpoonup}{\vphantom{a}\smash {#1}}} \)
\( \newcommand{\id}{\mathrm{id}}\) \( \newcommand{\Span}{\mathrm{span}}\)
( \newcommand{\kernel}{\mathrm{null}\,}\) \( \newcommand{\range}{\mathrm{range}\,}\)
\( \newcommand{\RealPart}{\mathrm{Re}}\) \( \newcommand{\ImaginaryPart}{\mathrm{Im}}\)
\( \newcommand{\Argument}{\mathrm{Arg}}\) \( \newcommand{\norm}[1]{\| #1 \|}\)
\( \newcommand{\inner}[2]{\langle #1, #2 \rangle}\)
\( \newcommand{\Span}{\mathrm{span}}\)
\( \newcommand{\id}{\mathrm{id}}\)
\( \newcommand{\kernel}{\mathrm{null}\,}\)
\( \newcommand{\range}{\mathrm{range}\,}\)
\( \newcommand{\RealPart}{\mathrm{Re}}\)
\( \newcommand{\ImaginaryPart}{\mathrm{Im}}\)
\( \newcommand{\Argument}{\mathrm{Arg}}\)
\( \newcommand{\norm}[1]{\| #1 \|}\)
\( \newcommand{\Span}{\mathrm{span}}\) \( \newcommand{\AA}{\unicode[.8,0]{x212B}}\)
\( \newcommand{\vectorA}[1]{\vec{#1}} % arrow\)
\( \newcommand{\vectorAt}[1]{\vec{\text{#1}}} % arrow\)
\( \newcommand{\vectorB}[1]{\overset { \scriptstyle \rightharpoonup} {\mathbf{#1}} } \)
\( \newcommand{\vectorC}[1]{\textbf{#1}} \)
\( \newcommand{\vectorD}[1]{\overrightarrow{#1}} \)
\( \newcommand{\vectorDt}[1]{\overrightarrow{\text{#1}}} \)
\( \newcommand{\vectE}[1]{\overset{-\!-\!\rightharpoonup}{\vphantom{a}\smash{\mathbf {#1}}}} \)
Learning Objectives
- Diagram the ABC model of flower development and identify the genes that control that development
Flower development is the process by which angiosperms produce a pattern of gene expression in meristems that leads to the appearance of a flower. A flower (also referred to as a bloom or blossom) is the reproductive structure found in flowering plants. There are three physiological developments that must occur in order for reproduction to take place:
- the plant must pass from sexual immaturity into a sexually mature state
- the apical meristem must transform from a vegetative meristem into a floral meristem or inflorescence
- the flowers individual organs must grow (modeled using the ABC model)
Flower Development
A flower develops on a modified shoot or axis from a determinate apical meristem (determinate meaning the axis grows to a set size). The transition to flowering is one of the major phase changes that a plant makes during its life cycle. The transition must take place at a time that is favorable for fertilization and the formation of seeds, hence ensuring maximal reproductive success. In order to flower at an appropriate time, a plant can interpret important endogenous and environmental cues such as changes in levels of plant hormones and seasonable temperature and photoperiod changes. Many perennial and most biennial plants require vernalization to flower.
Genetic Control of Flower Development
When plants recognize an opportunity to flower, signals are transmitted through florigen, which involves a variety of genes, including CONSTANS, FLOWERING LOCUS C and FLOWERING LOCUS T. Florigen is produced in the leaves in reproductively favorable conditions and acts in buds and growing tips to induce a number of different physiological and morphological changes.
From a genetic perspective, two phenotypic changes that control vegetative and floral growth are programmed in the plant. The first genetic change involves the switch from the vegetative to the floral state. If this genetic change is not functioning properly, then flowering will not occur. The second genetic event follows the commitment of the plant to form flowers. The sequential development of plant organs suggests that a genetic mechanism exists in which a series of genes are sequentially turned on and off. This switching is necessary for each whorl to obtain its final unique identity.

ABC Model of Flower Development
In the simple ABC model of floral development, three gene activities (termed A, B, and C-functions) interact to determine the developmental identities of the organ primordia (singular: primordium) within the floral meristem. The ABC model of flower development was first developed to describe the collection of genetic mechanisms that establish floral organ identity in the Rosids and the Asterids; both species have four verticils (sepals, petals, stamens and carpels), which are defined by the differential expression of a number of homeotic genes present in each verticil.
In the first floral whorl only A-genes are expressed, leading to the formation of sepals. In the second whorl both A- and B-genes are expressed, leading to the formation of petals. In the third whorl, B and C genes interact to form stamens and in the center of the flower C-genes alone give rise to carpels. For example, when there is a loss of B-gene function, mutant flowers are produced with sepals in the first whorl as usual, but also in the second whorl instead of the normal petal formation. In the third whorl the lack of B function but presence of C-function mimics the fourth whorl, leading to the formation of carpels also in the third whorl.
Most genes central in this model belong to the MADS-box genes and are transcription factors that regulate the expression of the genes specific for each floral organ.
- Flower development describes the process by which angiosperms (flowering plants) produce a pattern of gene expression in meristems that leads to the appearance of a flower; the biological function of a flower is to aid in reproduction.
- In order for flowering to occur, three developments must take place: (1) the plant must reach sexual maturity, (2) the apical meristem must transform from a vegetative meristem to a floral meristem, and (3) the plant must grow individual flower organs.
- These developments are initiated using the transmission of a complex signal known as florigen, which involves a variety of genes, including CONSTANS, FLOWERING LOCUS C and FLOWERING LOCUS T.
- The last development (the growth of the flower’s individual organs) has been modeled using the ABC model of flower development.
- Class A genes affect sepals and petals, class B genes affect petals and stamens, class C genes affect stamens and carpels.
- sepal : a part of an angiosperm, and one of the component parts of the calyx; collectively the sepals are called the calyx (plural calyces), the outermost whorl of parts that form a flower
- stamen : in flowering plants, the structure in a flower that produces pollen, typically consisting of an anther and a filament
- verticil : a whorl; a group of similar parts such as leaves radiating from a shared axis
- biennial : a plant that requires two years to complete its life cycle
- whorl : a circle of three or more leaves, flowers, or other organs, about the same part or joint of a stem
- apical meristem : the tissue in most plants containing undifferentiated cells (meristematic cells), found in zones of the plant where growth can take place at the tip of a root or shoot.
- angiosperm : a plant whose ovules are enclosed in an ovary
- perennial : a plant that is active throughout the year or survives for more than two growing seasons
- primordium : an aggregation of cells that is the first stage in the development of an organ
Contributions and Attributions
- Meristem. Provided by : Wikipedia. Located at : http://en.Wikipedia.org/wiki/Meristem . License : CC BY-SA: Attribution-ShareAlike
- meristem. Provided by : Wiktionary. Located at : en.wiktionary.org/wiki/meristem . License : CC BY-SA: Attribution-ShareAlike
- undifferentiated. Provided by : Wiktionary. Located at : en.wiktionary.org/wiki/undifferentiated . License : CC BY-SA: Attribution-ShareAlike
- apical. Provided by : Wiktionary. Located at : en.wiktionary.org/wiki/apical . License : CC BY-SA: Attribution-ShareAlike
- Provided by : Wikimedia. Located at : http://upload.wikimedia.org/Wikipedia/commons/d/d7/M%C3%A9rist%C3%A8me_coupe_zones_chiffres.png . License : CC BY-SA: Attribution-ShareAlike
- Provided by : Static Flckr. Located at : http://farm3.staticflickr.com/2441/5717178292_fd834167b1_o.jpg . License : CC BY: Attribution
- ABC model of flower development. Provided by : Wikipedia. Located at : en.Wikipedia.org/wiki/ABC_model_of_flower_development . License : CC BY-SA: Attribution-ShareAlike
- Flower. Provided by : Wikipedia. Located at : en.Wikipedia.org/wiki/Flower . License : CC BY-SA: Attribution-ShareAlike
- verticil. Provided by : Wiktionary. Located at : en.wiktionary.org/wiki/verticil . License : CC BY-SA: Attribution-ShareAlike
- primordium. Provided by : Wiktionary. Located at : en.wiktionary.org/wiki/primordium . License : CC BY-SA: Attribution-ShareAlike
- perennial. Provided by : Wiktionary. Located at : en.wiktionary.org/wiki/perennial . License : CC BY-SA: Attribution-ShareAlike
- apical meristem. Provided by : Wikipedia. Located at : en.Wikipedia.org/wiki/apical%20meristem . License : CC BY-SA: Attribution-ShareAlike
- sepal. Provided by : Wiktionary. Located at : en.wiktionary.org/wiki/sepal . License : CC BY-SA: Attribution-ShareAlike
- biennial. Provided by : Wiktionary. Located at : en.wiktionary.org/wiki/biennial . License : CC BY-SA: Attribution-ShareAlike
- stamen. Provided by : Wiktionary. Located at : en.wiktionary.org/wiki/stamen . License : CC BY-SA: Attribution-ShareAlike
- angiosperm. Provided by : Wiktionary. Located at : en.wiktionary.org/wiki/angiosperm . License : CC BY-SA: Attribution-ShareAlike
- whorl. Provided by : Wiktionary. Located at : en.wiktionary.org/wiki/whorl . License : CC BY-SA: Attribution-ShareAlike
- Provided by : Wikimedia. Located at : http://upload.wikimedia.org/Wikipedia/commons/e/ee/ABC_flower_development.svg . License : CC BY: Attribution
- Mature flower diagram. Provided by : Wikipedia. Located at : en.Wikipedia.org/wiki/File:Mature_flower_diagram.svg . License : CC BY-SA: Attribution-ShareAlike
- Search Menu
- Sign in through your institution
- Advance articles
- Darwin Reviews
- Special Issues
- Expert View
- Flowering Newsletter Reviews
- Technical Innovations
- Editor's Choice
- Virtual Issues
- Community Resources
- Reasons to submit
- Author Guidelines
- Peer Reviewers
- Submission Site
- Open Access
- About Journal of Experimental Botany
- About the Society for Experimental Biology
- Editorial Board
- Advertising and Corporate Services
- Journals Career Network
- Permissions
- Self-Archiving Policy
- Dispatch Dates
- Journal metrics
- Journals on Oxford Academic
- Books on Oxford Academic
Article Contents
Introduction, the origin of the agl6 subfamily pre-dates the common ancestor of gymnosperms and angiosperms, the evolutionary history of the agl6 subfamily in flowering plants reveals lineage duplications and losses, agl6 genes may have been recruited to regulate the unique features of monocot flowers, in petunia, the function of the euagl6 gene phagl6 is masked by its redundancy with the agl2 genes, agl6 and agl13 may have wide-ranging functions in arabidopsis flowering time, inflorescence, and flower development, the agl6 subfamily genes are expressed in both vegetative and reproductive tissues of gymnosperms and core eudicots, conclusion and perspective, supplementary data, acknowledgements.
- < Previous
Flower development: the evolutionary history and functions of the AGL6 subfamily MADS-box genes
Editor: Lars Hennig, Swedish University of Agricultural Sciences
- Article contents
- Figures & tables
- Supplementary Data
Ludovico Dreni, Dabing Zhang, Flower development: the evolutionary history and functions of the AGL6 subfamily MADS-box genes, Journal of Experimental Botany , Volume 67, Issue 6, March 2016, Pages 1625–1638, https://doi.org/10.1093/jxb/erw046
- Permissions Icon Permissions
AGL6 is an ancient subfamily of MADS-box genes found in both gymnosperms and angiosperms. Its functions remained elusive despite the fact that the MADS-box genes and the ABC model have been studied for >20 years. Nevertheless, recent discoveries in petunia, rice, and maize support its involvement in the ‘E’ function of floral development, very similar to the closely related AGL2 ( SEPALLATA ) subfamily which has been well characterized. The known functions of AGL6 span from ancient conserved roles to new functions acquired in specific plant families. The AGL6 genes are involved in floral meristem regulation, in floral organs, and ovule (integument) and seed development, and have possible roles in both male and female germline and gametophyte development. In grasses, they are also important for the development of the first whorl of the flower, whereas in Arabidopsis they may play additional roles before floral meristem formation. This review covers these recent insights and some other aspects that are not yet fully elucidated, which deserve more studies in the future.
One fundamental feature of plant evolution and adaptation to the land environment is the seed, serving as a protective ‘vessel’ for the new generation of sporophytes. The seed derives from an ovule with a fertilized embryo sac inside. Despite no continuous fossil record describing their earliest ancestors, it is commonly accepted that seed plants are a monophyletic group which originated ~310 million years ago (MYA) based on morphological and molecular evidence ( Schneider et al. , 2004 ). Among the existing seed plants, the ‘old’ term gymnosperms refers to a likely monophyletic group of four main taxa, namely Coniferophyta, Cycadophyta, Ginkgophyta, and Gnetophyta, the ovule/seed of which is neither developed nor protected by an ovary. Their sister lineage, referred to as angiosperms, or flowering plants, or Magnoliophyta, is a far larger taxon which arose ~140–198 MYA, between the Jurassic and Early Cretaceous ( Bell et al. , 2005 ; Moore et al. , 2007 ). During the Early and Late Cretaceous, flowering plants radiated exponentially to become the dominant land plant taxon.
The character which distinguishes flowering plants from gymnosperm is the formation of flowers, bisexual structures typically composed of reproductive organs surrounded by vegetative perianth organs. Stamens are the male reproductive organs that produce pollen. The presence of an ovary enclosing and protecting the ovule(s) at the centre of the flower is the other major distinctive character of this taxon. After the embryo sac is fertilized, the ovule starts to develop into a seed, and the surrounding ovary generates the fruit tissues.
Compared with the more ancient groups of land plants, it might not be surprising that such a drastic increase in structural complexity of reproductive organs in flowering plants required the recruitment of new molecular regulatory networks, which are orchestrated by transcription factors (TFs). The MADS-box TFs are indeed master regulators of these floral structures (as well as of nearly all the plant body and life cycle aspects). The way in which they genetically interact with one other to regulate flower development was first understood in two of the core eudicot plants, Arabidopsis thaliana (L.) Heynh. and Antirrhinum majus L., upon which the ABC model was built ( Coen and Meyerowitz, 1991 ). In the ABC model, a flower is composed of four concentric whorls bearing different kind of floral organs—sepals, petals, stamens, and carpels—sequentially arranged from the outer to the inner part of the flower. This floral structure is typical of core eudicots. There are three functional classes of TFs that regulate the organ identity in these four whorls: the A class genes alone specify sepal identity in the first whorl, and the combined activity of A and B genes is required for petal identity in the second whorl. Together, B and C genes initiate the establishment of stamen identity in the third whorl, and then C genes alone impose the termination and differentiation of the floral meristem (FM) into carpel. Later studies in petunia ( Petunia×hybrida hort. ex E.Vilm.) revealed more genes regulating ovule identity and development inside the carpel, where a D function was introduced into the initial model ( Angenent et al. , 1995 ; Colombo et al. , 1995 ). Finally, an E function was assigned to another class of genes, which is essential for the identity of all floral organs in combination with the A, B, C, and D genes ( Pelaz et al. , 2000 ; Theissen, 2001 ; Ditta et al. , 2004 ).
With the exception of the Arabidopsis A function gene APETALA2 ( AP2 ), all of the genes involved in the ABC(DE) model encode type II MADS-box TFs from the large MIKC C group ( Henschel et al. , 2002 ). Since several yeast two-hybrid interaction studies suggested that MADS-box proteins can form higher order complexes, it was proposed that the MADS-box proteins encoded by ABC(DE) genes form different combination of tetramers or quartets, resulting in a ‘quartet model’ that can explain the ABC model at the molecular level ( Honma and Goto, 2001 ; Theissen and Saedler, 2001 ; Favaro et al. , 2003 ). In vitro interaction studies suggest that DNA-bound MADS tetramers, or more properly double dimers, are the transcriptionally active form of the ‘ABC’ MADS-box TFs ( Melzer and Theissen, 2009 ; Melzer et al. , 2009 ; Smaczniak et al. , 2012 ; Jetha et al. , 2014 ).
Compared with core eudicots, the monocot flowers are significantly different, in particular in the external organs. Within monocots, rice ( Oryza sativa L.) and maize ( Zea mays L.) are the main models to investigate the development of grass inflorescences and flowers, and its molecular regulation (reviewed by Zhang and Yuan, 2014 ). In grasses, the flower consists of two opposite bract-like structures arranged in adjacent whorls, the lemma and the palea; the latter is mostly regarded as the true first whorl of the grass flower. In rice, the lemma and the palea are interlocked at their margins. The rice palea is morphologically similar to the lemma, but has only three vascular bundles instead of five, and two distinctive membranous, semi-transparent margins ( Prasad et al. , 2001 ; Prasad and Vijayraghavan, 2003 ). Enclosed by the lemma and the palea are two small lodicules in the second whorl, possibly homologous to petals; then six stamens in the third whorl and one ovary containing just one ovule in the centre. Maize produces two types of reproductive inflorescences, and in both of them spikelets are borne in pairs, with an upper and a lower floret in each spikelet. The apical male inflorescence (tassel) bears staminate flowers where pistil primordia are aborted during development. In the lateral female inflorescence (ear), the lower floret is aborted, whereas the upper floret aborts only the stamen primordia. Maize and rice flowers are similar, but in maize the lemma and palea have lost their protective function, and there are only three stamens.
The definition of A function, its conservation outside of the Brassicaceae family, as well as the homologous origin of the first floral whorl itself between different plant taxa are the subject of debate. Besides having a general function in the regulation of reproductive meristems, the SQUAMOSA ( SQUA ) subfamily MADS-box genes are considered to provide the A function in Brassicaceae at least ( Shan et al. , 2007 ). It is not clear if the second whorl organ of core eudicots and grass monocots has a homologous or an independent origin. Despite this, the B function seems quite well conserved in specifying its identity. Another hypothesis is that B function genes might have been recruited independently in dicots and monocots to play a similar role in the second whorl ( Whipple et al. , 2007 ). Current evidence reveals that the B, C, D, and E genes and their functions are highly conserved within flowering plants. In summary, the B function is provided by the DEFICIENS / GLOBOSA ( DEF/GLO ) subfamily, both C and D by the AGAMOUS ( AG ) subfamily, and E by the AGAMOUS-LIKE 2 ( AGL2 ; or SEPALLATA , SEP ) subfamily ( Zahn et al. , 2005a ; Kater et al. , 2006 ; Cui et al. , 2010 ; Ciaffi et al. , 2011 ; Dreni et al. , 2011 ; Li et al. , 2011b ; Yun et al. , 2013 ; Zhang et al. , 2013 ; Dreni and Kater, 2014 ). Studies conducted in petunia and Arabidopsis showed that the distinction of a true ‘D’ function from the C function is much more vague than initial assumptions, many AG C function genes being redundantly also involved in ovule development ( Pinyopich et al. , 2003 ; Heijmans et al. , 2012 ).
The MIKC C family includes several other genes which are not, or not yet, functionally included in the ABC model. Actually, 14 major subfamilies of MIKC C MADS-box genes have been identified in flowering plants, seven among which also exist in gymnosperms, which also possess the gymnosperm-specific GpMADS4 subfamily ( Becker and Theissen, 2003 ; Gramzow et al. , 2014 ). However, some of these subfamilies share a close relationship with the aforementioned DEF/GLO , AG , AGL2 , and SQUA involved in floral organ development. For example, the GGM13 ( B SISTER , B S ) and OsMADS32 lineages form a superclade together with the B function DEF/GLO . The former is probably involved in the development of ovule and seed integument in all seed plants ( Nesi et al. , 2002 ; Prasad and Ambrose, 2010 ; Prasad et al. , 2010 ; Mizzotti et al. , 2012 ; Yang et al. , 2012 ; Yin and Xue, 2012 ; Lee et al. , 2013 ; Lovisetto et al. , 2013 ; Nayar et al. , 2013 ), while the latter is found in monocots and in Amborella trichopoda Baill. ( Amborella Genome Project, 2013 ; Gramzow et al. , 2014 ) and, at least in rice, is important for proper floral organ identity ( Sang et al. , 2012 ; Wang et al. , 2015 ). The AGL12 subfamily is sister to the AG subfamily which regulates stamen, carpel, and ovule development, and FM determinacy. However, studies conducted in Arabidopsis showed a major function of AGL12 -like genes in both root development and floral transition ( Tapia-López et al. , 2008 ).
In this review, we focus on the AGL6 subfamily, sister to AGL2 ( SEP ), whose functions in flower development and plant reproduction have only been recently elucidated. Investigations by yeast two-hybrid screenings, as well as their close sequence similarity with AGL2 proteins, suggest that AGL6 TFs might form multimeric complexes with several ABCDE proteins. Not until 2009 did the direct evidence that they are indeed involved in floral organ identity and patterning emerge, thanks to various loss-of-function studies first in petunia, and shortly after in rice and maize. Furthermore, molecular studies in several other species also provide functional clues about this interesting and ancient group of TFs, which are seemingly ubiquitous in seed plants.
In published phylogenies, the AGL6 subfamily clusters as a sister group of the AGL2 subfamily with high confidence. Both of them, plus the SQUA subfamily, are included in the so-called AGL2/AGL6/SQUA superclade (reviewed by Becker and Theissen, 2003 ). A recent discovery is that the FLOWERING LOCUS C ( FLC ) subfamily, a key player in the vernalization response in both eudicots and grasses, also belongs to this superclade. The possibility that the TOMATO MADS 3 subfamily ( TM3 or SOC1 -like, from SUPPRESSOR OF CONSTANS 1 ) may share the same origin requires further validation ( Ruelens et al. , 2013 ). Through a combination of genome synteny and phylogenetic reconstructions, a single MIKC C -type MADS-box gene has been shown at the origin of the whole superclade, which underwent a tandem duplication before the most recent common ancestor (MRCA) of seed plants. This ancestral tandem duplication can be considered the earliest event of the series which gave birth to both the SQUA–FLC sister subfamilies and the AGL2–AGL6 sister subfamilies. These four subfamilies are all represented in angiosperms, but only AGL6 has been maintained in the gymnosperm lineage.
The close phylogenetic relationship between AGL2 and AGL6 subfamilies is reflected by both the sequence and structure similarities of their encoded proteins. AGL6 proteins possess a divergent C-terminus with two short, but highly conserved regions, which are referred to as AGL6-I and AGL6-II motifs, respectively ( Ohmori et al. , 2009 ). Both AGL6 motifs share consistent similarity to the corresponding SEP I and SEP II motifs that are typically found in the C-terminus of AGL2 proteins ( Zahn et al. , 2005a ), and could function as the transcriptional activation motifs of the floral identity quartets ( Malcomber and Kellogg, 2005 ; Zahn et al. , 2005a ). Indeed, in the rice AGL6 proteins OsMADS6 and OsMADS17, the C-terminal region spanning the two motifs induces strong transcriptional activation in yeast ( Ohmori et al. , 2009 ). This, together with the functional studies described below, led to the hypothesis that AGL6 subfamily genes mainly have an E function in floral development, similar to the well-characterized AGL2 , SEP -like genes.
To date, the most informative phylogenetic analyses of AGL6 subfamily genes have been conducted by Viaene et al. (2010) and Li et al (2010) . Due to the release of several new genomes and transcriptomes recently, we are able to update our understanding of this subfamily in angiosperms, and in particular in monocots, from an evolutionary perspective ( Fig. 1 ; Supplementary Figs S1–S5 at JXB online).

ML phylogenetic tree of the AGL6 subfamily. The tree was built from 100 subreplicates using gene coding sequences from gymnosperms, monocots, and core eudicots. The relevant previously reported lineage duplications are indicated with a black star. The scale bar indicates the number of nucleotide changes per site. Accession numbers are provided in Supplementary Table S1.
The subfamilies of MADS-box genes regulating floral development in angiosperms generally underwent a number of interesting duplication events which occurred before their radiation into the extant taxa, where they were subsequently maintained. In particular, many well-documented lineage duplications are observed in both core eudicots and grasses ( Kramer et al. , 1998 , 2004 ; Litt and Irish, 2003 ; Malcomber and Kellogg, 2005 ; Zahn et al. , 2005a, b ; Shan et al. , 2007 ; Viaene et al. , 2010 ; Ciaffi et al. , 2011 ; Airoldi and Davies, 2012 ; Vekemans et al. , 2012 ; Ruelens et al. , 2013 ). Most of these duplications, if not all, derived from whole-genome duplication (WGD) events, probably because of the gene balance hypothesis ( Edger and Pires, 2009 ; Airoldi and Davies, 2012 ).
Compared with these examples, the angiosperm AGL6 did not expand much in paralogue lineages, despite more than one gene existing in this subfamily in most species. In general, where AGL6 paralogue lineages are found, at least one of them is poorly conserved ( Fig. 1 ). Based on the phylogenetic reconstructions, a duplication in the AGL6 subfamily may have occurred before the MRCA of gymnosperms ( Fig. 1 ) ( Zahn et al. , 2005a ; Li et al. , 2010 ), but probably a single AGL6 lineage has been maintained in the putative polyploid ancestor of angiosperms. Within angiosperms, Viaene and collaborators (2010) found a lineage duplication in magnoliids and two paralogue clades in core eudicots, which are named AGL6 -like and euAGL6 . The former is frequently lost (also in Brassicaceae), as we could find it in less than half of the available rosid genomes, and it corresponds to the legume PsMADS3 clade ( Hecht et al. , 2005 ; Wong et al. , 2013 ). In asterids, in agreement with previous analysis, AGL6 -like sequences could be found only in Roridulaceae and Actinidiaceae, which are placed in the basal order Ericales. Furthermore, the function of core eudicot AGL6 -like members is totally obscure. Interestingly, all the AGL6-like proteins show several amino acid substitutions in highly conserved residues, including the MADS-box domain, and in the C-terminus the AGL6 motifs I and II are nearly lost (Supplementary Fig. S1), suggesting that these genes underwent a process of neofunctionalization, or maybe pseudogenization. Conversely, the euAGL6 clade is strongly conserved, and is only absent in the current flax ( Linum usitatissimum L.) genome assembly and available transcriptomic data from the Linum genus, where only AGL6 -like genes are found. The two Arabidopsis euAGL6 genes, AGL6 and AGL13 ( Fig. 1 ), are assumed to be derived from the WGD (named as α) that preceded the diversification of Brassicaceae ( Vision et al. , 2000 ; Bowers et al. , 2003 ).
Our survey of sequences from monocots revealed a more complex scenario than the previous assumptions. Phylogenetic analysis by maximum likelihood (ML), parsimony, and Neighbor–Joining (NJ) methods identified four main, well-defined monocot clades, named AGL6 -I to AGL6 -IV, plus the only sequence available from the order Liliales, the Alstroemeria ligtu L. AGL6 homologue. However, due to low bootstrapping and unclear relationship in the three analyses, we collapsed their basal nodes ( Fig. 1 ; Supplementary Figs S2, S3).
The wide clade AGL6 -I is commelinid specific (Arecales, Zingiberales, Poales), and in grasses it further divides into two well-defined branches that here we name, after their founder members, ZAG3 (which is often referred to in the literature as the OsMADS6 clade) and OsMADS17 . Based on the current sequence and genomes, the AGL6 -I/ ZAG3 clade is conserved in all grasses. Despite first being described as specific for the Oryza genus, we showed that the AGL6 -I/ OsMADS17 clade was lost in the MRCA of Pooideae but retained in Panicoideae ( Fig. 1 ). In Panicoideae, the OsMADS17 clade is found in the genera Panicum , Setaria , and Sorghum , the latter of which has lost the AGL6 motif II. In the ancient tetraploid maize, the OsMADS17 clade was lost; however, there are two OsMADS6 paralogues, zag3 and zag5 , which will be discussed in the next section.
The clade AGL6 -II is represented by a few sequences from Asparagales and bamboo grasses, as already reported by Viaene et al. (2010) , whereas the new clade AGL6 -III is only represented by orchids. Finally, the new AGL6 -IV clade is supported by a limited number of sequences from orchids in Asparagales and from the commelinids African oil palm ( Elaeis guineensis Jacq., Arecales) and banana ( Musa acuminata Colla, Zingiberales), suggesting that this clade is also poorly conserved.
Our detailed analysis of the moso bamboo, Phyllostachys edulis (Carrière) J. Houzeau (synonym Phyllostachys heterocycla Carrière), genome ( Peng et al. , 2013 ) shows that it has all three clades, namely AGL6 -I/ ZAG3 , AGL6 -I/ OsMADS17 , and AGL6 -II. This strongly supports the hypothesis of an ancient divergence of clades I and II, where clade I might have been retained only in commelinids and clade II might have experienced multiple independent losses during monocot evolution, in particular in commelinids. Furthermore, it reveals that all three clades were represented in the grass MRCA and that AGL6 -II was lost only after the divergence of Bambusoideae from the main grass lineages.
In summary, in our phylogenetic analysis, the monocot AGL6 subfamily divides into four main branches, two of which are reported for the first time in this study. They probably derived from putative duplications which occurred before the radiation of Asparagales, Liliales, and Commelinids. However, further studies are required to determine the precise relationship and the origin of these clades. Previously, a single early duplication event had been hypothesized in the monocot AGL6 subfamily ( Viaene et al. , 2010 ), which could fit well with the single WGD (τ) that has been predicted before the separation of commelinids ( Jiao et al. , 2014 ). However, a simple duplication event does not seem to be enough to explain the complex AGL6 phylogeny that we reveal in monocots, and this analysis is limited by the current lack of whole-genome sequences, especially outside commelinids.
In contrast, the further separation of clade AGL6-I in the grass-specific OsMADS17 and ZAG3 clades matches very well with the most recent WGD (ρ) which occurred before the MRCA of extant grasses ( Paterson et al. , 2004 ; Wang et al. , 2005 ; Jiao et al. , 2014 ). In rice, OsMADS6 and OsMADS17 reside in the long arms of chromosomes 2 and 4, respectively, which are highly syntenic to each other ( Thiel et al. , 2009 ). Furthermore, we have found consistent microsynteny around the two loci (not shown). How early this WGD occurred in the grass ancestor lineage is not clear. A sequence from Joinvillea ascendens Brongn. & Gris, which belongs to a grass sister lineage, clusters strongly with the ZAG3 clade in the NJ analysis, but not in ML and parsimony ( Fig. 1 ; Supplementary Figs S1, S2).
The expression of AGL6 subfamily genes in the perianth differs within flowering plant taxa ( Fig. 2 ). Its expression in the FM, carpel primordium, and ovule integument seems ancestral and is highly conserved in grasses, but not in stamens. In grasses, its expression is conserved in the palea, which is regarded as an evolutionary novelty of the family due to its link to the acquisition of new functions in the first floral whorl. The expression of the AGL6 subfamily genes in the second whorl is conserved in grasses and their close relatives ( Reinheimer and Kellogg, 2009 ).

Comparison of the expression domain of AGL6 genes from representative species. (A) Pinus radiata PrMADS3 is expressed in ovuliferous scale primordia (arrows) in stage 2 female seed cone buds. (B) In the vegetative dwarf shoot buds of Pinus radiata , PrMADS3 is expressed in the initiating needle primordia (arrows). (C) In the young flower bud of petunia, PhAGL6 is most expressed in petal and ovary primordia, but also in anthers. (D) In the petunia ovary at anthesis, PhAGL6 is mainly expressed in ovule primordia. (E) In Arabidopsis floral buds, AGL6 is expressed at the base of all floral whorls, whereas in the Arabidopsis ovule (F), it is expressed in the endothelium. (G) In rice, OsMADS6 expression starts between spikelet stage Sp3 and Sp4, in the FM and palea primordium. (H) During mid stages of rice flower development, OsMADS6 expression is strong in the lodicules; in the palea it becomes restricted to the margin tissues. Inside the carpel, strong expression is found in the ovule integuments, but it is also reported in the nucellus area where the MMC differentiates. The drawings and expression patterns are inspired by: Mouradov et al. (1998) ; Favaro et al. (2002) ; Pelucchi et al. (2002) ; Ohmori et al. (2009) ; Rijpkema et al. (2009) ; Schauer et al. (2009) ; Koo et al. (2010) ; Li et al. (2010) ; and Zhang et al. (2010) . ap, apical meristem; sc, sterile cataphylls; o, developing ovary; c, carpel/carpel wall; a, anther; p, petal; s, sepal; pl, placenta; ov, ovule; en, endothelium; sl, sterile lemma; le, lemma; pa, palea; fm, floral meristem; lo, lodicule.
The rice OsMADS6 gene, also called MOSAIC FLORAL ORGANS1 ( MFO1 ; Ohmori et al. , 2009 ), is probably the most well characterized AGL6 subfamily gene in seed plants. It was isolated and characterized for its ability to interact with most of the other rice AGL2/AGL6/SQUA TFs (OsMADS1, 5, 45/7, 24/8, 14, 15, 17, and 18) >15 years ago ( Moon et al. , 1999 ); however, functional characterization studies did not take place until 2009. There was some inconsistency in the numbering of published mutant alleles ( Ohmori et al. , 2009 ; Li et al. , 2010 ; Zhang et al. , 2010 ), and here we follow the nomenclature given by Duan and co-workers (2012) ( Table 1 ). OsMADS6 is first expressed in the FM at stage Sp3 of spikelet development ( Fig. 2 ), following the stages proposed by Ikeda et al. (2004) and Itoh et al. (2005) . After the formation of the lemma primordium, it is expressed in the emerging palea primordium ( Ohmori et al. , 2009 ; Li et al. , 2010 ). Its expression is also detected in developing palea and lodicules, and continues until the formation of the pistil primordium in the FM, where it soon becomes restricted to the ovule integuments ( Favaro et al. , 2002 ; Pelucchi et al. , 2002 ; Ohmori et al. , 2009 ). Noticeably, OsMADS6 is not expressed in developing stamen primordia.
List of published rice OsMADS6 mutant alleles
The nomenclature follows that used by Duan et al. (2012).
Strong osmads6 mutant alleles show floral defects in all tissues where the gene is expressed ( Ohmori et al. , 2009 ; Li et al. , 2010 ). No defects are found in the lemma, whereas the marginal tissues of the palea are completely missing, resulting in the disappearance of the well-interlocked structure which normally exists between the lemma and palea ( Fig. 3 ). Furthermore, the palea vascular bundles increased from three to five or six. Thus the palea is in fact homeotically converted into lemma in severe osmads6 alleles. Lodicules were converted in elongated glume-like structures, and additional glume-like and lodicule/stamen chimeric organs appeared between whorls 2 and 3 ( Fig. 3 ). The defects in the FM lead to a decrease in the average number of stamens in the third whorl. Depending on the mutant allele, two or more carpels or even new spikelets were often observed in the fourth whorl, suggesting a partial loss of FM determinacy, while ovules partially lost their identity within carpels, thus leading to strong female sterility ( Li et al. , 2010 ).

Flower phenotype of the rice osmads6-1 strong mutant. (A) Wild-type mature flower before anthesis. Bottom right: a papery, semi-transparent marginal tissue characterizes the palea margin (arrows). (B) In osmads6-1 , the flower is enlarged as the palea is converted into a lemma-like organ, not interlocked with the lemma. Bottom right: the glume interlocking is lost because the marginal tissue is totally missing from the palea margins of the mutant (arrows). (C) Second, third, and fourth whorl organs of a rice wild-type flower. Second whorl lodicules are indicated with a red arrow. (D) In osmads6-1 , lodicules are mostly transformed into glume-like organs (red arrow), and stamen/lodicule/glume chimeric organs develop in the third whorl. Scale bar=0.5mm. a, anther; l, lemma; lp, lemma-like palea; p, palea; sl, sterile lemma.
Some of the floral defects found in osmads6 mutants resemble those caused by mutants in the AGL2 -like gene OsMADS1 ( Jeon et al. , 2000 ; Agrawal et al. , 2005 ). OsMADS1 and OsMADS6 are able to form heterodimers ( Moon et al. , 1999 ). Interestingly, the osmads1 and osmads6 phenotypes increase dramatically in forms of double mutants ( Ohmori et al. , 2009 ; Li et al. , 2010 ), suggesting that OsMADS1 and OsMADS6 might have partially redundant functions in regulating spikelet meristem determinacy and its transition to FM, as well as floral patterning. Further evidence for the redundancy between OsMADS1 and OsMADS6 is that they are both required for the proper expression of a common set of floral genes, such as B, C, and E floral homeotic MADS-box genes, especially during early floral stages ( Chen et al. , 2006 ; Li et al. , 2011a ; Hu et al. , 2015 ). Additionally, they are both required for the proper expression of genes involved in hormonal signalling and homeostasis like OsMGH3 , even though at least for OsMADS1 the regulation of this gene seems to be indirect ( Prasad et al. , 2005 ; Zhang et al. , 2010 ; Yadav et al. , 2011 ).
Finally, OsMADS6 and OsMADS1 are likely to be targets of similar regulatory pathways. The expression of both is enhanced in RNAi knock-down lines of the LDB-like TF gene OsIG1 , another regulator of floral development ( Zhang et al. , 2015 ), and they are also putative targets of the Polycomb Repressor Complex 2 (PRC2), which seems essential for the regulation of FM determinacy and fate in rice ( Conrad et al. , 2014 ).
In osmads6 , the homeotic conversion of lodicules into membranous or glume-like organs is similar to the effect of B class loss-of-function mutants ( Nagasawa et al. , 2003 ; Prasad and Vijayraghavan, 2003 ; Yao et al. , 2008 ), despite both AP3 and PI homologues still being expressed in the abnormal floral organs of the osmads6 mutant ( Ohmori et al. , 2009 ). Nevertheless, OsMADS6 has been shown to interact with the rice DEF/GLO heterodimers (OsMADS2–OsMADS16 and OsMADS4–OsMADS16) to form nuclear-localized ternary complexes ( Seok et al. , 2010 ), suggesting that OsMADS6 is an important subunit of the B class complex which specifies lodicule identity in the second whorl. OsMADS6 seems to be required to repress the AP1/SQUA-like genes OsMADS14 and OsMADS15 in the second whorl ( Ohmori et al. , 2009 ).
As OsMADS6 is not expressed during the differentiation of the third whorl, it is not likely to be required for the formation of the B–C complex to determine stamen identity. Indeed, stamen identity is not affected completely in osmads6 mutants, and the change in stamen number and the appearance of ectopic third whorl chimeric organs can be attributed to a role for OsMADS6 in FM regulation and identity. However, OsMADS6 expression was reported in the tapetum and in the male germline ( Reinheimer and Kellogg, 2009 ; Tang et al. , 2010 ; Zhang et al. , 2010 ), implying possible function in male gametogenesis.
In the fourth whorl, the defects found in osmads6 in terms of FM determinacy, and gynoecium and ovule development corroborate the role of OsMADS6 as an interactor of the D function protein OsMADS13 ( Favaro et al. , 2002 ). In the ovule nucellus, OsMADS6 expression was reported in the zone of the differentiating megaspore mother cell (MMC) ( Zhang et al. , 2010 ). Interestingly, the weak mutant allele mfo1-2 ( Ohmori et al. , 2009 ) produced normal carpels but sometimes defective ovules with the outgrowth of outer integument and the absence of an embryo sac, which was replaced by an abnormally proliferated nucellus. Furthermore, in Brachiaria brizantha (Hochst. ex A.Rich.) Stapf, a tropical forage grass characterized by facultative aposporic apomixis, the OsMADS6 homologue BbrizAGL6 is expressed differentially in the nucellus of apomictic and sexual plants. In fact, it seems to mark the formation of the MMC in sexual plants and the aposporic initial (AI) precursor cells in apomictic plants ( Guimarães et al. , 2013 ). Therefore, it will be interesting to test whether grass AGL6 -like genes are involved in the differentiation of the MMC.
The function of OsMADS6 in gynoecium is unlikely to be restricted to its formation, as it continues to be expressed for several days after pollination in the developing kernel, along with its paralogue OsMADS17 ( Arora et al. , 2007 ). It was reported that a minority of the osmads6 mutant pistils are fertile; however, the kernels produced were rounder and defective in filling, with a reduced starch content and increased protein fraction ( Zhang et al. , 2010 ). It was not clarified whether those kernel phenotypes are under maternal control, or if they depend on some OsMADS6 functions in the progeny tissues. OsMADS6 is able to interact with the B SISTER TF OsMADS29 ( Nayar et al. , 2014 ) which has an essential conserved function for the development of the kernel, where they appear to be largely co-expressed ( Yang et al. , 2012 ; Nayar et al. , 2013 ).
Interestingly, a new recessive allele of OsMADS6 , namely osmads6-5 (or the ‘ lemmata ’ mutant), was reported recently ( Duan et al. , 2012 ). This allele exhibits phenotypical abnormalities which are markedly different from those of any other osmads6 mutant. In its homozygotes, the lemma remains unaffected. Similar to the other osmads6 mutants, the palea loses its identity and is converted into a lemma-like organ, but with up to 10 vascular bundles. Notably, inside of the first whorl, the indeterminate FM continuously produces new lemma-like organs; hence the name ‘ lemmata ’ is given to this mutant. Therefore, because of this novel phenotype, the authors argued that none of the previous mutants is a null allele, which, however, is questionable ( Table 1 ). The osmads6-5 mutant itself cannot be considered as a knock-out, as it is still able to transcribe a chimeric RNA with partial OsMADS6 ORFs. Despite the authors performing a careful analysis to exclude possible semi-dominant or co-suppression effects from this transcript, we assume that this very interesting allele might have caused some unexpected molecular effect which warrants further studies.
In comparison with OsMADS6, its paralogue OsMADS17 ( Moon et al. , 1999 ) is far less well studied. The expression patterns of OsMADS6 and OsMADS17 are largely overlapping, and the transcript level of OsMADS17 is increased in osmads6 mutants ( Ohmori et al. , 2009 ). Using an RNAi approach, Ohmori and colleagues (2009) observed that OsMADS17 could play minor redundant roles that are only visible when the function of OsMADS6 is severely compromised. We believe that the global elucidation of the rice AGL6 subfamily genes requires stable knock-out mutants of OsMADS17 , and its double mutants with osmads6 .
In maize, the OsMADS17 clade was lost whereas the ZAG3 clade contains the two duplicated genes zag3 and zag5 ( Mena et al. , 1995 ). The bearded-ear ( bde ) mutant is caused by the loss of function of zag3 ( Thompson et al. , 2009 ). In the mutant tassels, spikelets produce more florets with more floral organs, which are often silks. In the ear of the mutant, spikelets also contain multiple florets, which in turn produce more palea–lemma-like organs, while the ovaries are mostly sterile with extra silks. The bde mutants affect the upper and lower floret meristems differentially.
To our knowledge, no mutant of AGL6 subfamily genes has yet been reported in non-grass monocots. In some species, the number of AGL6 -like genes is expanded. For example, we found three loci from the African oil palm and a large expansion of five loci in the banana genome ( Fig. 1 ). The genome of the orchid Phalaenopsis equestris (Schauer) Rchb.f. has three AGL6 -like genes plus a putative pseudogene ( Cai et al. , 2014 ). The authors argued that the expansion of AGL2 , AGAMOUS , DEF/AP3 , and AGL6 clades might be the origin of the unique evolutionary novelties found in orchids. In line with this, the expression profiling of MADS-box genes conducted in the related species Phalaenopsis aphrodite Rchb.f. led to the proposition of a modified ABCDE model, where PaAGL6-1 is being expressed only in the lip ( Su et al. , 2013 ).
Only one euAGL6 gene is known in petunia, the PETUNIA MADS BOX GENE4 ( pMADS4 ) ( Tsuchimoto et al. , 2000 ) which has been renamed as Petunia hybrida AGL6 ( PhAGL6 ) ( Rijpkema et al. , 2009 ). This gene is specifically expressed in flowers, where its transcripts were detected at high levels first in the petal primordium and in the early ovary primordium, and later in the ovule primordia ( Fig. 2 ). At significantly lower levels, the transcript was found even in the other floral organs. Despite this, phagl6 , the first null mutant reported for a plant AGL6 -like gene, did not show obvious phenotypes in flowers. PhAGL6 is largely co-expressed with some AGL2 subfamily genes, such as FBP2 ( Ferrario, 2003 ; Rijpkema et al. , 2009 ). In fbp2 single mutants, the corolla was converted to green sepal-like organs at the edges of the petals and along the main veins at the abaxial side ( Vandenbussche et al. , 2003 ). Indeed the fbp2 phenotype was dramatically enhanced in a fbp2 phagl6 double mutant, resulting in completely green petals and reduced corolla size. Moreover, the stamens of phagl6 fbp2 double mutants were also affected, showing sepal/petal-like structures and occasionally stigma-like structures on top of the anthers ( Rijpkema et al. , 2009 ). As further evidence of redundancy between petunia AGL6 and AGL2 genes, mutation of another AGL2 -like gene, FBP5 , further enhanced the phagl6 fbp2 double mutant phenotype, causing a more severe conversion of petals and stamens into sepal- or leaf-like organs ( Rijpkema et al. , 2009 ). The strong expression of PhAGL6 in the ovary and ovule primordia, together with the ability of its protein product to interact with C and D proteins, suggests that PhAGL6 may also have an E-like function in the fourth whorl. However, this is not supported by mutant analysis, suggesting complete redundancy with other AGL2 genes ( Rijpkema et al. , 2009 ). Despite the function of AGL6 in petunia and Arabidopsis gynoecium not yet being clear, the fact that Arabidopsis AGL6 is one of the few MIKC C -type proteins able to interact with some type I MADS proteins ( de Folter et al. , 2005 ) led to the hypothesis that they might be involved together in the development of the ovule and gametophyte ( Rijpkema et al. , 2009 ).
The Arabidopsis genome has two euAGL6 genes, AGL6 ( Ma et al. , 1991 ) and AGL13 ( Rounsley et al. , 1995 ).
AGL6 is expressed during the vegetative phase at a similar level in different growth stages, under both long- and short-day conditions, suggesting that AGL6 is regulated in a photoperiod-independent manner ( Yoo et al. , 2011b ). AGL6 activation tagged mutants showed early flowering phenotypes, which are associated with the down-regulation of the floral repressor FLC and of its homologues MAF4 and MAF5 , and with the up-regulation of the floral promoter FT . These findings indicate that AGL6 is a strong floral promoter ( Yoo et al. , 2011b ). Moreover, AGL6 activation tagged mutants have altered leaf movement and expression of core oscillator genes. This suggests that AGL6 can play a role in control of the circadian clock, although knock-down alleles suggested that there might be redundancy with other MADS TFs ( Yoo et al. , 2011a ).
Through the analysis of epistatic natural alleles, it was shown that AGL6 specifically affects stem branching by promoting axillary bud formation ( Huang et al. , 2012 ). AGL6 expression was also found in the abaxial and proximal region of cauline leaf primordia, in the cryptic bracts subtending newly initiated floral primordia 6 d after transfer to long days, and later at the base of all floral organs ( Fig. 2 ) ( Koo et al. , 2010 ). Expression of a fusion of the AGL6 genomic sequence to the strong transcriptional activation domain VP16 under the control of the AGL6 promoter ( gAGL6::VP16 ), led to the formation of ectopic organs on most flower pedicels, which were initially bract like. Together, these findings suggest a role for AGL6 in suppressing the outgrowth of the cryptic bracts. Furthermore, the ectopic organs became increasingly carpel and stamen like in later developing flowers, and finally flowers were completely replaced by staminoid bracts, suggesting an additional role for AGL6 in floral organ identity. This effect was caused by a LFY -independent activation of B and C homeotic genes ( Koo et al. , 2010 ). Furthermore, the ectopic expression of either AGL6 ( 35S:AGL6 ) or a fusion of AGL6 with the EAR transcriptional repression domain ( 35S:AGL6::EAR ) dramatically accelerated flowering time, suggesting the role of AGL6 in regulating flowering time by repressing floral repressors ( Koo et al. , 2010 ).
In flowers, AGL6 and AGL13 are co-expressed only in the developing chalaza of the ovule. AGL6 is also expressed in the endothelium ( Fig. 2 ), whereas AGL13 is expressed in the innermost anther wall layer, the tapetum. In addition, an AGL13 reporter line showed activity in the vasculature underlying the developing shoot apical meristem ( Schauer et al. , 2009 ).
Recently, the characterization of reporter lines where β-glucuronidase (GUS) is driven by an AGL13 genomic region comprising the putative regulatory elements ( Hsu et al. , 2014 ) suggested a broader expression profile in the FM, anther, pollen up to stage 12, and ovules compared with the more cell type-specific pattern reported by Schauer and colleagues (2009) . In 35S:AGL13 RNAi transgenic lines, about half of the ovules were aborted at early stages, and pollen was also affected, showing aberrations in the exine patterning of the outer wall; however, half of the pollen was still viable and able to germinate and fertilize the egg cell. Further genetic analysis showed that after meiosis and tetrad formation, the development of the two pollen grains of each tetrad carrying the RNAi transgene was arrested ( Hsu et al. , 2014 ). In order to create dominant loss-of-function mutants, AGL13 was fused to the SRDX suppressing motif and expressed ectopically. This resulted in similar phenotypes compared with RNAi experiments, suggesting that AGL13 mainly functions as a transcriptional activator. In addition, the most severe 35S:AGL13::SRDX lines produced considerably shorter sepals, petals, and anthers that were similar to 35S:SEP2::SRDX plants ( Hsu et al. , 2014 ), indicating that AGL13 and SEP2 may have a similar function and target similar genes during flower development. Furthermore, both AGL13 and SEP3 proteins can enhance the nuclear localization efficiency of AG. Fluorescence resonance energy transfer (FRET) experiments indicated that AGL13 and AG can form quartet-like complexes. Finally, the AG–AGL13 heterodimer could also interact with the AP3–PI heterodimer ( Hsu et al. , 2014 ), which may be functional against known target genes of AG in the anther, such as SPL/NZZ ( Ito et al. , 2004 ).
The AGL6 genes have been reported also to be expressed in the vegetative tissues of gymnosperms, but not within monocots, magnoliids, and Ranunculales. However, as they are frequently expressed in the vegetative organs in core eudicots, it has been proposed that their vegetative expression was lost in angiosperms but regained in core eudicots ( Viaene et al. , 2010 ).
In gymnosperms, the AGL6 subfamily divides into two clades ( Fig. 1 ), which are represented by DAL1 and DAL14 in Norway spruce [ Picea abies (L.) H.Karst.]. In Pinus radiata D.Don, PrMADS2 and PrMADS3 are orthologous to DAL14 and DAL1 , respectively. Current data strongly support that, at least in these two conifers, the DAL14/PrMADS2 clade is active exclusively in male and female reproductive tissues, whereas the DAL1/PrMADS3 clade is active in both reproductive and vegetative shoots ( Tandre et al. , 1995 ; Mouradov et al. , 1998 ; Carlsbecker et al. , 2004 ). In female cones, all these genes are expressed in the ovuliferous scales and ovule primordia, with some differences in the precise pattern and timing. DAL1 has been proposed as a possible mediator of the juvenile-to-adult transition in Norway spruce ( Carlsbecker et al. , 2004 ). In P. radiata , the PrMADS3 transcript was precisely localized in the peripheral zone of the vegetative dwarf shoot buds where the needle primordia are differentiating, and then in the needle primordia ( Fig. 2 ) ( Mouradov et al. , 1998 ). However in Gnetum gnemon L. (Gnetophyta), the expression of both the AGL6 -like genes GGM9 ( DAL1/PrMADS3 clade) and GGM11 ( DAL14/PrMADS2 clade) has been reported only in male and female cones to date ( Winter et al. , 1999 ).
In core eudicots where the AGL6 subfamily has two major clades, the expression of AGL6 -like genes is highest in vegetative tissues, and in tendrils of grape vine ( Vitis vinifera L.), whereas euAGL6 genes are predominantly expressed in floral tissues and occasionally in vegetative tissues ( Viaene et al. , 2010 ).
To sum up, the AGL6-like proteins show a conserved and broad spectrum of interactions with other MIKC C proteins in petunia ( Rijpkema et al. , 2009 ), Arabidopsis ( de Folter et al. , 2005 ; Hsu et al. , 2014 ), and rice ( Moon et al. , 1999 ; Favaro et al. , 2002 ; Seok et al. , 2010 ; Nayar et al. , 2014 ), which is also very similar to the interactome of the AGL2-like proteins.
At least in petunia and rice, there is a significant functional redundancy between the AGL6 and AGL2 factors ( Ohmori et al. , 2009 ; Rijpkema et al. , 2009 ; Li et al. , 2010 ). Regarding this, it is worth mentioning that single recessive loss-of-function mutants of Arabidopsis AGL6 genes do not show abnormal phenotypes, which were mostly caused by the expression of its dominant activated or repressed forms ( Koo et al. , 2010 ; Hsu et al. , 2014 ). Now that targeted mutagenesis, in particular CRISPR/CAS9, is becoming a standard technique, real null mutants for these genes in Arabidopsis should be generated, in order to test their function and the possible genetic interactions with SEP genes.
Therefore, based on the molecular characterization and mutant analyses carried out in petunia, rice, and maize, we can add the AGL6 genes to the E function of the ABC model, as was first proposed and proven by Rijpkema and co-workers (2009) . For example, their functions are evident in the second and third whorl of petunia, and very probably also in the fourth whorl. In rice, OsMADS6 is active in the first whorl, which probably represents a neofunctionalization of AGL6 genes of grasses, and in the second and fourth whorls. Based on the expansion in terms of gene number and on expression profiles, it has been proposed that AGL6 genes might have been important for the evolution of the unique flower features of orchids. In Arabidopsis, data suggest that they may also function during the vegetative phase, in flowering time regulation, and in shaping the inflorescence architecture. In addition, a number of AGL6 subfamily genes from monocots, eudicots, and gymnosperms have been overexpressed in Arabidopsis and tobacco, suggesting similar functions (reviewed by Rijpkema et al. , 2009 ; Viaene et al. , 2010 ).
If AGL6 genes play just the same roles as AGL2 , it would be expected that these two ancestral clades are not both essential for the plant and one or the other should frequently have been lost in different angiosperm lineages. In constrast, there seems to be a strong positive selection pressure on AGL6 in known flowering plant genomes, with the possible exception of flax, suggesting that their functions cannot be identical to those of the AGL2 subfamily genes. Furthermore, the AGL2 subfamily expanded in several conserved clades during the evolution of angiosperms, whereas the expansion of the AGL6 subfamily was more limited. However, these questions are yet to be addressed thoroughly. Several studies pointed to a possible correlation of the AGL6 genes with the development of male and female sporogenous tissues, and also with the first stages of male and female gametophyte development. Moreover, at least in Arabidopsis, AGL6 is a possible interactor of some type I MADS proteins ( de Folter et al. , 2005 ), which is unusual for MIKC C -type MADS proteins. Indeed, many type I MADS proteins are known players in female gametophyte, and embryo and endosperm development (reviewed by Masiero et al. , 2011 ).
The strong expression of Arabidopsis AGL6 in the ovule endothelium suggests an unknown role in the development of this important cell layer. It will be interesting to test whether AGL6 forms a MADS complex with SEEDSTICK (STK) and ARABIDOPSIS B SISTER (ABS), which are essential for endothelium development ( Nesi et al. , 2002 ; Mizzotti et al. , 2012 ). For the less conserved AGL6 -like clade of core eudicots, which are frequently expressed in vegetative tissues, no function has been yet assigned. Thus, the functional significance and evolutionary origins of AGL6 genes still await clarification.
Due to the similarity and close phylogenetic relationship of AGL6 and AGL2 proteins, it has been speculated for a long time that AGL6 might mediate the formation of floral identity quartets in gymnosperms, as they are the only possible players of the E function in these plants, where all the AGL6 sister clades are missing. However, the notion that gymnosperms and angiosperms possess essentially a similar set of MIKC C TF subfamilies does not automatically imply that MADS tetramers must form in the same way in all seed plants. Notably, the gene duplication event that led to the formation of the DEF and GLO subfamilies within B class genes occurred in the angiosperm lineage after its separation from the gymnosperms lineage ( Zahn et al. , 2005b ), and the establishment of a DEF/GLO obligate heterodimer occurred even later in the most evolved angiosperm taxa ( Winter et al. , 2002 ; Melzer et al. , 2014 ). Moreover, studies conducted in G. gnemon MADS proteins supported their ability to form DNA-bound tetramers made only of B and C subunits, or only C (but not only B) subunits, which does not require the E proteins acting like glue ( Melzer et al. , 2010 ; Wang et al. , 2010 ). On the other hand, whether these complexes observed in vitro are also able to trigger proper transcription of their target genes without the need for the putative AGL6 and AGL2 transcriptional activation motifs, is not yet clear ( Wang et al. , 2010 ). Regarding these aspects, the AGL6 members are still far less understood than other MIKC TFs and deserve more studies in the future.
Supplementary data are available at JXB online.
Figure S1. Alignment of eudicot AGL6-like, eudicot euAGL6 (orange), and grass ZAG3
clade proteins.
Figure S2. Phylogeny of AGL6 subfamily genes in seed plants, constructed by the parsimony method.
Figure S3. Phylogeny of AGL6 subfamily genes in seed plants, constructed by the NJ method.
Figure S4. Multifasta protein whole sequence alignment.
Figure S5. Multifasta codon alignment used to infer the phylogeny.
Table S1. Gene accession numbers.
We thank Junyan Li for editing the paper, and Professor Wen-Chieh Tsai (Institute of Tropical Plant Sciences, National Cheng Kung University, Tainan, Taiwan) for providing full AGL6 subfamily sequences from P. equestris. Our research on rice E function genes is supported by the National Natural Science Foundation of China (NSFC) (31230051), the NSFC Research Fund for International Young Scientists (31550110198), the Programme of Introducing Talents of Discipline to Universities (111 Project, B14016), and the China Postdoctoral Science Foundation (2014M560328). LD is supported by a Marie Skłodowska-Curie Global Fellowship (Horizon 2020 call H2020-MSCA-IF-2014, project 661678 - RiceStyle). We apologize to the authors of papers that we could not discuss in this manuscript, due to space constraints.
Agrawal GK Abe K Yamazaki M Miyao A Hirochika H . 2005 . Conservation of the E-function for floral organ identity in rice revealed by the analysis of tissue culture-induced loss-of-function mutants of the OsMADS1 gene . Plant Molecular Biology 59 , 125 –1 35 .
Google Scholar
Airoldi CA Davies B . 2012 . Gene duplication and the evolution of plant MADS-box transcription factors . Journal of Genetics and Genomics 39 , 157 – 165 .
Amborella Genome Project . 2013 . The Amborella genome and the evolution of flowering plants . Science 342 , 1241089 .
Angenent GC Franken J Busscher M van Dijken A van Went JL Dons HJ van Tunen AJ . 1995 . A novel class of MADS box genes is involved in ovule development in petunia . The Plant Cell 7 , 1569 – 1582 .
Arora R Agarwal P Ray S Singh AK Singh VP Tyagi AK Kapoor S . 2007 . MADS-box gene family in rice: genome-wide identification, organization and expression profiling during reproductive development and stress . BMC Genomics 8 , 242 .
Becker A Theissen G . 2003 . The major clades of MADS-box genes and their role in the development and evolution of flowering plants . Molecular Phylogenetics and Evolution 29 , 464 – 489 .
Bell CD Soltis DE Soltis PS . 2005 . The age of the angiosperms: a molecular timescale without a clock . Evolution 59 , 1245 – 1258 .
Google Preview
Bowers JE Chapman BA Rong J Paterson AH . 2003 . Unraveling angiosperms genome evolution by phylogenetic analysis of chromosomal duplications events . Nature 422 , 433 – 438 .
Cai J Liu X Vanneste K et al. . 2014 . The genome sequence of the orchid Phalaenopsis equestris . Nature Genetics 47 , 65 – 72 .
Carlsbecker A Tandre K Johanson U Englund M Engström P . 2004 . The MADS-box gene DAL1 is a potential mediator of the juvenile-to-adult transition in Norway spruce (Picea abies) . The Plant Journal 40 , 546 – 557 .
Chen ZX Wu JG Ding WN Chen HM Wu P Shi CH . 2006 . Morphogenesis and molecular basis on naked seed rice, a novel homeotic mutation of OsMADS1 regulating transcript level of AP3 homologue in rice . Planta 223 , 882 – 890 .
Ciaffi M Paolacci AR Tanzarella OA Porceddu E . 2011 . Molecular aspects of flower development in grasses . Sexual Plant Reproduction 24 , 247 – 282 .
Coen ES Meyerowitz EM . 1991 . The war of the whorls: genetic interactions controlling flower development . Nature 353 , 31 – 37 .
Colombo L Franken J Koetje E van Went J Dons HJ Angenent GC van Tunen AJ . 1995 . The petunia MADS box gene FBP11 determines ovule identity . The Plant Cell 7 , 1859 – 1868 .
Conrad LJ Khanday I Johnson C Guiderdoni E An G Vijayraghavan U Sundaresan V . 2014 . The polycomb group gene EMF2B is essential for maintenance of floral meristem determinacy in rice . The Plant Journal 80 , 883 – 94 .
Cui R Han J Zhao S Su K Wu F Du X Xu Q Chong K Theissen G Meng Z . 2010 . Functional conservation and diversification of class E floral homeotic genes in rice (Oryza sativa) . The Plant Journal 61 , 767 – 781 .
de Folter S Immink RGH Kieffer M et al. . 2005 . Comprehensive interaction map of the Arabidopsis MADS Box transcription factors . The Plant Cell 17 , 1424 – 1433 .
Ditta G Pinyopich A Robles P Pelaz S Yanofsky MF . 2004 . The SEP4 gene of Arabidopsis thaliana functions in floral organ and meristem identity . Current Biology 14 , 1935 – 1940 .
Dreni L Kater MM . 2014 . MADS reloaded: evolution of the AGAMOUS subfamily genes . New Phytologist 201 , 717 – 732 .
Dreni L Pilatone A Yun D Erreni S Pajoro A Caporali E Zhang D Kater MM . 2011 . Functional analysis of all AGAMOUS subfamily members in rice reveals their roles in reproductive organ identity determination and meristem determinacy . The Plant Cell 23 , 2850 – 2863 .
Duan Y Xing Z Diao Z et al. . 2012 . Characterization of Osmads6-5, a null allele, reveals that OsMADS6 is a critical regulator for early flower development in rice (Oryza sativa L.) . Plant Molecular Biology 80 , 429 – 442 .
Edger PP Pires JC . 2009 . Gene and genome duplications: the impact of dosage-sensitivity on the fate of nuclear genes . Chromosome Research 17 , 699 – 717 .
Favaro R Immink RGH Ferioli V Bernasconi B Byzova M Angenent GC Kater M Colombo L . 2002 . Ovule-specific MADS-box proteins have conserved protein–protein interactions in monocot and dicot plants . Molecular Genetics and Genomics 268 , 152 – 159 .
Favaro R Pinyopich A Battaglia R Kooiker M Borghi L Ditta G Yanofsky M Kater M Colombo L . 2003 . MADS-box protein complexes control carpel and ovule development in Arabidopsis . The Plant Cell 15 , 2603 – 2611 .
Ferrario S . 2003 . The MADS box gene FBP2 is required for SEPALLATA function in Petunia . The Plant Cell 15 , 914 – 925 .
Gramzow L Theissen G . 2010 . A hitchhiker’s guide to the MADS world of plants . Genome Biology 11 , 214 .
Gramzow L Weilandt L Theißen G . 2014 . MADS goes genomic in conifers: towards determining the ancestral set of MADS-box genes in seed plants . Annals of Botany 114 , 1407 – 29 .
Guimarães LA Diva DM Masiero S Resentini F Gomes ACMM Silveira ÉD Florentino LH Rodrigues JCM Colombo L Vera VT . 2013 . BbrizAGL6 is differentially expressed during embryo sac formation of apomictic and sexual Brachiaria brizantha plants . Plant Molecular Biology Reporter 31 , 1397 – 1406 .
Hecht V Foucher F Ferrándiz C et al. . 2005 . Conservation of Arabidopsis flowering genes in model legumes . Plant Physiology 137 , 1420 – 34 .
Heijmans K Ament K Rijpkema AS Zethof J Wolters-Arts M Gerats T Vandenbussche M . 2012 . Redefining C and D in the Petunia ABC . The Plant Cell 24 , 2305 – 2317 .
Henschel K Kofuji R Hasebe M Saedler H Münster T Theissen G . 2002 . Two ancient classes of MIKC-type MADS-box genes are present in the moss Physcomitrella patens . Molecular Biology and Evolution 19 , 801 – 814 .
Honma T Goto K . 2001 . Complexes of MADS-box proteins are sufficient to convert leaves into floral organs . Nature 409 , 525 – 529 .
Hsu WH Yeh TJ Huang KY Li JY Chen HY Yang CH . 2014 . AGAMOUS-LIKE13, a putative ancestor for the E functional genes, specifies male and female gametophyte morphogenesis . The Plant Journal 77 , 1 – 15 .
Hu Y Liang W Yin C et al. . 2015 . Interactions of OsMADS1 with floral homeotic genes in rice flower development . Molecular Plant 8 , 1366 – 1384 .
Huang X Effgen S Meyer RC Theres K Koornneef M . 2012 . Epistatic natural allelic variation reveals a function of AGAMOUS-LIKE6 in axillary bud formation in Arabidopsis . The Plant Cell 24 , 2364 – 2379 .
Ikeda K Sunohara H Nagato Y . 2004 . Developmental course of inflorescence and spikelet in rice . Breeding Science 54 , 147 – 156 .
Ito T Wellmer F Yu H Das P Ito N Alves-Ferreira M Riechmann JL Meyerowitz EM . 2004 . The homeotic protein AGAMOUS controls microsporogenesis by regulation of SPOROCYTELESS . Nature 430 , 356 – 360 .
Itoh JI Nonomura KI Ikeda K Yamaki S Inukai Y Yamagishi H Kitano H Nagato Y . 2005 . Rice plant development: from zygote to spikelet . Plant and Cell Physiology 46 , 23 – 47 .
Jeon JS Jang S Lee S et al. . 2000 . leafy hull sterile1 is a homeotic mutation in a rice MADS box gene affecting rice flower development . The Plant Cell 12 , 871 – 84 .
Jetha K Theißen G Melzer R . 2014 . Arabidopsis SEPALLATA proteins differ in cooperative DNA-binding during the formation of floral quartet-like complexes . Nucleic Acids Research 42 , 10927 – 10942 .
Jiao Y Li J Tang H Paterson AH . 2014 . Integrated syntenic and phylogenomic analyses reveal an ancient genome duplication in monocots . The Plant Cell 26 , 2792 – 2802 .
Kater MM Dreni L Colombo L . 2006 . Functional conservation of MADS-box factors controlling floral organ identity in rice and Arabidopsis . Journal of Experimental Botany 57 , 3433 – 3444 .
Koo SC Bracko O Park MS et al. . 2010 . Control of lateral organ development and flowering time by the Arabidopsis thaliana MADS-box gene AGAMOUS-LIKE6 . The Plant Journal 62 , 807 – 816 .
Kramer EM Dorit RL Irish VF . 1998 . Molecular evolution of genes controlling petal and stamen development: duplication and divergence within the APETALA3 and PISTILLATA MADS-box gene lineages . Genetics 149 , 765 – 783 .
Kramer EM Jaramillo MA Di Stilio VS . 2004 . Patterns of gene duplication and functional evolution during the diversification of the AGAMOUS subfamily of MADS box genes in angiosperms . Genetics 166 , 1011 – 1023 .
Lee DS Chen LJ Li CY et al. . 2013 . The Bsister MADS gene FST determines ovule patterning and development of the zygotic embryo and endosperm . PLoS One 8 , e58748 .
Li H Liang W Hu Y Zhu L Yin C Xu J Dreni L Kater MM Zhang D . 2011 a . Rice MADS6 interacts with the floral homeotic genes SUPERWOMAN1, MADS3, MADS58, MADS13, and DROOPING LEAF in specifying floral organ identities and meristem fate . The Plant Cell 23 , 2536 – 2552 .
Li H Liang W Jia R Yin C Zong J Kong H Zhang D . 2010 . The AGL6-like gene OsMADS6 regulates floral organ and meristem identities in rice . Cell Research 20 , 299 – 313 .
Li H Liang W Yin C Zhu L Zhang D . 2011 b . Genetic interaction of OsMADS3, DROOPING LEAF, and OsMADS13 in specifying rice floral organ identities and meristem determinacy . Plant Physiology 156 , 263 – 274 .
Litt A Irish VF . 2003 . Duplication and diversification in the APETALA1/FRUITFULL floral homeotic gene lineage: implications for the evolution of floral development . Genetics 165 , 821 – 833 .
Lovisetto A Guzzo F Busatto N Casadoro G . 2013 . Gymnosperm B-sister genes may be involved in ovule/seed development and, in some species, in the growth of fleshy fruit-like structures . Annals of Botany 112 , 535 – 544 .
Ma H Yanofsky MF Meyerowitz EM . 1991 . AGL1–AGL6, an Arabidopsis gene family with similarity to floral homeotic and transcription factor genes . Genes and Development 5 , 484 – 495 .
Malcomber ST Kellogg EA . 2005 . SEPALLATA gene diversification: brave new whorls . Trends in Plant Science 10 , 427 – 435 .
Masiero S Colombo L Grini PE Schnittger A Kater MM . 2011 . The emerging importance of type I MADS box transcription factors for plant reproduction . The Plant Cell 23 , 865 – 872 .
Melzer R Harter A Rumpler F Kim S Soltis PS Soltis DE Theissen G . 2014 . DEF- and GLO-like proteins may have lost most of their interaction partners during angiosperm evolution . Annals of Botany 114 , 1431 – 1443 .
Melzer R Theissen G . 2009 . Reconstitution of ‘floral quartets’ in vitro involving class B and class E floral homeotic proteins . Nucleic Acids Research 37 , 2723 – 2736 .
Melzer R Verelst W Theissen G . 2009 . The class E floral homeotic protein SEPALLATA3 is sufficient to loop DNA in ‘floral quartet’-like complexes in vitro . Nucleic Acids Research 37 , 144 – 157 .
Melzer R Wang YQ Theissen G . 2010 . The naked and the dead: the ABCs of gymnosperm reproduction and the origin of the angiosperm flower . Seminars in Cell and Developmental Biology 21 , 118 – 28 .
Mena M Mandel MA Lerner DR Yanofsky MF Schmidt RJ . 1995 . A characterization of the MADS-box gene family in maize . The Plant Journal 8 , 845 – 54 .
Mizzotti C Mendes MA Caporali E Schnittger A Kater MM Battaglia R Colombo L . 2012 . The MADS box genes SEEDSTICK and ARABIDOPSIS Bsister play a maternal role in fertilization and seed development . The Plant Journal 70 , 409 – 20 .
Moon YH Kang HG Jung JY Jeon JS Sung SK An G . 1999 . Determination of the motif responsible for interaction between the rice APETALA1/AGAMOUS-LIKE9 family proteins using a yeast two-hybrid system . Plant Physiology 120 , 1193 – 1204 .
Moore MJ Bell CD Soltis PS Soltis DE . 2007 . Using plastid genome-scale data to resolve enigmatic relationships among basal angiosperms . Proceedings of the National Academy of Sciences, USA 104 , 19363 – 19368 .
Mouradov A Glassick T V. Hamdorf BA Murphy LC Marla SS Yang Y Teasdale RD . 1998 . Family of MADS-Box genes expressed early in male and female reproductive structures of monterey pine . Plant Physiology 117 , 55 – 62 .
Nagasawa N Miyoshi M Sano Y Satoh H Hirano H Sakai H Nagato Y . 2003 . SUPERWOMAN1 and DROOPING LEAF genes control floral organ identity in rice . Development 130 , 705 – 718 .
Nayar S Kapoor M Kapoor S . 2014 . Post-translational regulation of rice MADS29 function: homodimerization or binary interactions with other seed-expressed MADS proteins modulate its translocation into the nucleus . Journal of Experimental Botany 65 , 5339 – 5350 .
Nayar S Sharma R Tyagi AK Kapoor S . 2013 . Functional delineation of rice MADS29 reveals its role in embryo and endosperm development by affecting hormone homeostasis . Journal of Experimental Botany 64 , 4239 – 4253 .
Nesi N Debeaujon I Jond C Stewart AJ Jenkins GI Caboche M Lepiniec L . 2002 . The TRANSPARENT TESTA16 locus encodes the ARABIDOPSIS BSISTER MADS domain protein and is required for proper development and pigmentation of the seed coat . The Plant Cell 14 , 2463 – 2479 .
Ohmori S Kimizu M Sugita M Miyao A Hirochika H Uchida E Nagato Y Yoshida H . 2009 . MOSAIC FLORAL ORGANS1, an AGL6-like MADS box gene, regulates floral organ identity and meristem fate in rice . The Plant Cell 21 , 3008 – 3025 .
Paterson AH Bowers JE Chapman BA . 2004 . Ancient polyploidization predating divergence of the cereals, and its consequences for comparative genomics . Proceedings of the National Academy of Sciences, USA 101 , 9903 – 9908 .
Pelaz S Ditta GS Baumann E Wisman E Yanofsky MF . 2000 . B and C floral organ identity functions require SEPALLATA MADS-box genes . Nature 405 , 200 – 203 .
Pellegrini L Tan S Richmond TJ . 1995 . Structure of serum response factor core bound to DNA . Nature 376 , 490 – 498 .
Pelucchi N Fornara F Favalli C Masiero S Lago C Pè EM Colombo L Kater MM . 2002 . Comparative analysis of rice MADS-box genes expressed during flower development . Sexual Plant Reproduction 15 , 113 – 122 .
Peng Z Lu Y Li L et al. . 2013 . The draft genome of the fast-growing non-timber forest species moso bamboo (Phyllostachys heterocycla) . Nature Genetics 45 , 456 – 461 , 461e1 – 2 .
Pinyopich A Ditta GS Savidge B Liljegren SJ Baumann E Wisman E Yanofsky MF . 2003 . Assessing the redundancy of MADS-box genes during carpel and ovule development . Nature 424 , 85 – 88 .
Prasad K Ambrose BA . 2010 . Shaping up the fruit: control of fruit size by an Arabidopsis B-sister MADS-box gene . Plant Signaling and Behavior 5 , 899 – 902 .
Prasad K P . S Kumar S Kushalappa K Vijayraghavan U . 2001 . Ectopic expression of rice OsMADS1 reveals a role in specifying the lemma and palea, grass floral organs analogous to sepals . Development Genes and Evolution 211 , 281 – 290 .
Prasad K Parameswaran S Vijayraghavan U . 2005 . OsMADS1, a rice MADS-box factor, controls differentiation of specific cell types in the lemma and palea and is an early-acting regulator of inner floral organs . The Plant Journal 43 , 915 – 928 .
Prasad K Vijayraghavan U . 2003 . Double-stranded RNA interference of a rice PI/GLO paralog, OsMADS2, uncovers its second-whorl-specific function in floral organ patterning . Genetics 165 , 2301 – 2305 .
Prasad K Zhang X Tobón E Ambrose BA . 2010 . The Arabidopsis B-sister MADS-box protein, GORDITA, represses fruit growth and contributes to integument development . The Plant Journal 62 , 203 – 214 .
Reinheimer R Kellogg EA . 2009 . Evolution of AGL6-like MADS box genes in grasses (Poaceae): ovule expression is ancient and palea expression is new . The Plant Cell 21 , 2591 – 2605 .
Rijpkema AS Zethof J Gerats T Vandenbussche M . 2009 . The petunia AGL6 gene has a SEPALLATA-like function in floral patterning . The Plant Journal 60 , 1 – 9 .
Rounsley SD Ditta GS Yanofsky MF . 1995 . Diverse roles for MADS box genes in Arabidopsis development . The Plant Cell 7 , 1259 – 1269 .
Ruelens P de Maagd RA Proost S Theißen G Geuten K Kaufmann K . 2013 . FLOWERING LOCUS C in monocots and the tandem origin of angiosperm-specific MADS-box genes . Nature Communications 4 , 2280 .
Sang X Li Y Luo Z et al. . 2012 . CHIMERIC FLORAL ORGANS1, encoding a monocot-specific MADS box protein, regulates floral organ identity in rice . Plant Physiology 160 , 788 – 807 .
Schauer SE Schlüter PM Baskar R Gheyselinck J Bolaños A Curtis MD Grossniklaus U . 2009 . Intronic regulatory elements determine the divergent expression patterns of AGAMOUS-LIKE6 subfamily members in Arabidopsis . The Plant Journal 59 , 987 – 1000 .
Schneider H Schuettpelz E Pryer KM Cranfill R Magallón S Lupia R . 2004 . Ferns diversified in the shadow of angiosperms . Nature 428 , 553 – 557 .
Seok HY Park HY Park JI Lee YM Lee SY An G Moon YH . 2010 . Rice ternary MADS protein complexes containing class B MADS heterodimer . Biochemical and Biophysical Research Communications 401 , 598 – 604 .
Shan H Zhang N Liu C Xu G Zhang J Chen Z Kong H . 2007 . Patterns of gene duplication and functional diversification during the evolution of the AP1/SQUA subfamily of plant MADS-box genes . Molecular Phylogenetics and Evolution 44 , 26 – 41 .
Smaczniak C Immink RGH Muiño JM et al. . 2012 . Characterization of MADS-domain transcription factor complexes in Arabidopsis flower development . Proceedings of the National Academy of Sciences, USA 109 , 1560 – 1565 .
Su CL Chen WC Lee AY Chen CY Chang YCA Chao YT Shih MC . 2013 . A modified ABCDE model of flowering in orchids based on gene expression profiling studies of the moth orchid Phalaenopsis aphrodite . PLoS One 8 , e80462 .
Tandre K Albert VA Sundås A Engström P . 1995 . Conifer homologues to genes that control floral development in angiosperms . Plant Molecular Biology 27 , 69 – 78 .
Tang X Zhang ZY Zhang WJ Zhao XM Li X Zhang D Liu QQ Tang WH . 2010 . Global gene profiling of laser-captured pollen mother cells indicates molecular pathways and gene subfamilies involved in rice meiosis . Plant Physiology 154 , 1855 – 70 .
Tapia-López R García-Ponce B Dubrovsky JG Garay-Arroyo A Pérez-Ruíz RV Kim S-H Acevedo F Pelaz S Alvarez-Buylla ER . 2008 . An AGAMOUS-related MADS-box gene, XAL1 (AGL12), regulates root meristem cell proliferation and flowering transition in Arabidopsis . Plant Physiology 146 , 1182 – 1192 .
Theissen G . 2001 . Development of floral organ identity: stories from the MADS house . Current Opinion in Plant Biology 4 , 75 – 85 .
Theissen G Saedler H . 2001 . Plant biology. Floral quartets . Nature 409 , 469 – 471 .
Thiel T Graner A Waugh R Grosse I Close TJ Stein N . 2009 . Evidence and evolutionary analysis of ancient whole-genome duplication in barley predating the divergence from rice . BMC Evolutionary Biology 9 , 209 .
Thompson BE Bartling L Whipple C Hall DH Sakai H Schmidt R Hake S . 2009 . bearded-ear encodes a MADS box transcription factor critical for maize floral development . The Plant Cell 21 , 2578 – 2590 .
Tsuchimoto S Mayama T van der Krol A Ohtsubo E . 2000 . The whorl-specific action of a petunia class B floral homeotic gene . Genes to Cells 5 , 89 – 99 .
Vandenbussche M Zethof J Souer E Koes R Tornielli GB Pezzotti M Ferrario S Angenent GC Gerats T . 2003 . Toward the analysis of the petunia MADS box gene family by reverse and forward transposon insertion mutagenesis approaches: B, C, and D floral organ identity functions require SEPALLATA-like MADS box genes in petunia . The Plant Cell 15 , 2680 – 2693 .
Vekemans D Proost S Vanneste K Coenen H Viaene T Ruelens P Maere S Van de Peer Y Geuten K . 2012 . Gamma paleohexaploidy in the stem lineage of core eudicots: significance for MADS-box gene and species diversification . Molecular Biology and Evolution 29 , 3793 – 3806 .
Viaene T Vekemans D Becker A Melzer S Geuten K . 2010 . Expression divergence of the AGL6 MADS domain transcription factor lineage after a core eudicot duplication suggests functional diversification . BMC Plant Biology 10 , 148 .
Vision TJ Brown DG Tanksley SD . 2000 . The origins of genomic duplications in Arabidopsis . Science 290 , 2114 – 2117 .
Wang YQ Melzer R Theissen G . 2010 . Molecular interactions of orthologues of floral homeotic proteins from the gymnosperm Gnetum gnemon provide a clue to the evolutionary origin of ‘floral quartets’ . The Plant Journal 64 , 177 – 190 .
Wang H Zhang L Cai Q et al. . 2015 . OsMADS32 interacts with PI-like proteins and regulates rice flower development . Journal of Integrative Plant Biology 57 , 504 – 513 .
Wang X Shi X Hao B Ge S Luo J . 2005 . Duplication and DNA segmental loss in the rice genome: implications for diploidization . New Phytologist 165 , 937 – 946 .
Whipple CJ Zanis MJ Kellogg EA Schmidt RJ . 2007 . Conservation of B class gene expression in the second whorl of a basal grass and outgroups links the origin of lodicules and petals . Proceedings of the National Academy of Sciences, USA 104 , 1081 – 1086 .
Winter KU Becker A Münster T Kim JT Saedler H Theissen G . 1999 . MADS-box genes reveal that gnetophytes are more closely related to conifers than to flowering plants . Proceedings of the National Academy of Sciences, USA 96 , 7342 – 7347 .
Winter KU Weiser C Kaufmann K Bohne A Kirchner C Kanno A Saedler H Theissen G . 2002 . Evolution of class B floral homeotic proteins: obligate heterodimerization originated from homodimerization . Molecular Biology and Evolution 19 , 587 – 596 .
Wong CE Singh MB Bhalla PL . 2013 . Novel members of the AGAMOUS LIKE 6 subfamily of MIKCC-type MADS-box genes in soybean . BMC Plant Biology 13 , 105 .
Yadav SR Khanday I Majhi BB Veluthambi K Vijayraghavan U . 2011 . Auxin-responsive OsMGH3, a common downstream target of OsMADS1 and OsMADS6, controls rice floret fertility . Plant and Cell Physiology 52 , 2123 – 2135 .
Yang X Wu F Lin X Du X Chong K Gramzow L Schilling S Becker A Theißen G Meng Z . 2012 . Live and let die—the B(sister) MADS-box gene OsMADS29 controls the degeneration of cells in maternal tissues during seed development of rice (Oryza sativa) . PLoS One 7 , e51435 .
Yao SG Ohmori S Kimizu M Yoshida H . 2008 . Unequal genetic redundancy of rice PISTILLATA orthologs, OsMADS2 and OsMADS4, in lodicule and stamen development . Plant and Cell Physiology 49 , 853 – 857 .
Yin LL Xue HW . 2012 . The MADS29 transcription factor regulates the degradation of the nucellus and the nucellar projection during rice seed development . The Plant Cell 24 , 1049 – 1065 .
Yoo SK Hong SM Lee JS Ahn JH . 2011 a . A genetic screen for leaf movement mutants identifies a potential role for AGAMOUS-LIKE 6 (AGL6) in circadian-clock control . Molecules and Cells 31 , 281 – 287 .
Yoo SK Wu X Lee JS Ahn JH . 2011 b . AGAMOUS-LIKE 6 is a floral promoter that negatively regulates the FLC/MAF clade genes and positively regulates FT in Arabidopsis . The Plant Journal 65 , 62 – 76 .
Yun D Liang W Dreni L Yin C Zhou Z Kater MM Zhang D . 2013 . OsMADS16 genetically interacts with OsMADS3 and OsMADS58 in specifying floral patterning in rice . Molecular Plant 6 , 743 – 756 .
Zahn LM Kong H Leebens-Mack JH Kim S Soltis PS Landherr LL Soltis DE Depamphilis CW Ma H . 2005 a . The evolution of the SEPALLATA subfamily of MADS-box genes: a preangiosperm origin with multiple duplications throughout angiosperm history . Genetics 169 , 2209 – 23 .
Zahn LM Leebens-Mack J DePamphilis CW Ma H Theissen G . 2005 b . To B or not to B a flower: the role of DEFICIENS and GLOBOSA orthologs in the evolution of the angiosperms . Journal of Heredity 96 , 225 – 240 .
Zhang D Yuan Z . 2014 . Molecular control of grass inflorescence development . Annual Review of Plant Biology 65 , 553 – 78 .
Zhang D Yuan Z An G Dreni L Hu J Kater MM . 2013 . Panicle development . In: Zhang Q Wing RA , eds. Genetics and genomics of rice, plant genetics and genomics: crops and models 5 . New York : Springer Science+Business Media , 279 – 295 .
Zhang J Nallamilli BR Mujahid H Peng Z . 2010 . OsMADS6 plays an essential role in endosperm nutrient accumulation and is subject to epigenetic regulation in rice (Oryza sativa) . The Plant Journal 64 , 604 – 617 .
Zhang J Tang W Huang Y Niu X Zhao Y Han Y Liu Y . 2015 . Down-regulation of a LBD-like gene, OsIG1, leads to occurrence of unusual double ovules and developmental abnormalities of various floral organs and megagametophyte in rice . Journal of Experimental Botany 66 , 99 – 112 .
Author notes
Email alerts, citing articles via.
- Recommend to your Library
Affiliations
- Online ISSN 1460-2431
- Print ISSN 0022-0957
- Copyright © 2024 Society for Experimental Biology
- About Oxford Academic
- Publish journals with us
- University press partners
- What we publish
- New features
- Open access
- Institutional account management
- Rights and permissions
- Get help with access
- Accessibility
- Advertising
- Media enquiries
- Oxford University Press
- Oxford Languages
- University of Oxford
Oxford University Press is a department of the University of Oxford. It furthers the University's objective of excellence in research, scholarship, and education by publishing worldwide
- Copyright © 2024 Oxford University Press
- Cookie settings
- Cookie policy
- Privacy policy
- Legal notice
This Feature Is Available To Subscribers Only
Sign In or Create an Account
This PDF is available to Subscribers Only
For full access to this pdf, sign in to an existing account, or purchase an annual subscription.
Genetics of Flower Development
Cite this chapter.
- K. V. Krishnamurthy 5 &
- Bir Bahadur 6
4517 Accesses
1 Citations
The flower is a unique feature of flowering plants. Recent research on molecular biology has indicated that a flower is the result of expression and interplay of several genes operating in a sequence. At least four pathways trigger floral evocation: temperature pathway (vernalization and ambient pathways), light quality pathway, photoperiod pathway and gibberellin pathway. Production of different floral organs and the decision of boundary between them are controlled by several genes whose activity can be explained by ABC + DE model, as well as by the quartet model. This article also explains the genetic basis of all floral variations including floral symmetry. The genetic basis of termination of the floral meristem is also explained. Finally, the chapter discusses evolution of flower and floral organs from a genetic perspective.
This is a preview of subscription content, log in via an institution to check access.
Access this chapter
- Available as PDF
- Read on any device
- Instant download
- Own it forever
- Available as EPUB and PDF
- Compact, lightweight edition
- Dispatched in 3 to 5 business days
- Free shipping worldwide - see info
- Durable hardcover edition
Tax calculation will be finalised at checkout
Purchases are for personal use only
Institutional subscriptions
Similar content being viewed by others
Flower Development: Open Questions and Future Directions
Flower development in the asterid lineage.
Flower Development in the Solanaceae
Abe M, Kobayashi Y, Yamamoto S, Yamaguchi A, Daimon Y, Ichinoki H, Ikeda Y, Notaguchi M, Goto K, Araki T (2005) FD, a bZIP protein mediating signals from the floral pathway integrator FT at the shoot apex. Science 309:1052–1056
Article CAS PubMed Google Scholar
Achard P, Herr A, Baulcombe DC, Harberd NP (2004) Modulation of floral development by gibberellins-regulated micro-RNA. Development 131:3357–3365
Alvarez-Buylla ER, Ambrose BA, Flores-Sandoval E, Vergara-Silva F, Englund M, Garay-Arroyo A, Garcia-Ponce B, de la Torre-Barcena E, Espinosa-Matias S, Martinez E et al (2010) B-function expression in the flower center underlies the homeotic phenotype of Lacandonia schismatica (Triuridaceae). Plant Cell 22:3543–3559
Article CAS PubMed Central PubMed Google Scholar
Amasino R (2010) Seasonal and developmental timing of flowering. Plant J 61:1001–1013
Bernier G (1988) The control of floral evocation and morphogenesis. Annu Rev Plant Physiol Plant Mol Biol 39:175–219
Article Google Scholar
Bernier G, Périlleux C (2005) A physiological overview of the genetics of flowering time control. Plant Biotechnol J 3:3–16
Bernier G, Havelange A, Houssa C, Petitijean A, Lejeune P (1993) Physiological signals that induce flowering. Plant Cell 5:1147–1155
Bommert P, Satoh-Nagasawa N, Jackson D, Hirano H-Y (2005) Genetics and evolution of inflorescence and flower development in grasses. Plant Cell Physiol 46:69–78
Boss PK, Bastow RM, Mylne JS, Dean C (2004) Multiple pathways in the decision to flower: enabling, promoting, and resetting. Plant Cell 16:518–531
Bowman JL (1997) Evolutionary conservation of angiosperm flower development at the molecular and genetic levels. J Biosci 22:515–527
Bowman JL, Smyth DR, Meyerowitz EM (2012) The ABC model of flower development: then and now. Development 139:4095–4098
Chen X (2004) A microRNA as a translational repressor of APETALA2 in Arabidopsis flower development. Science 303:2022–2025
Ciaffi M, Paolacci AR, Tanzarella OA, Porceddu E (2011) Molecular aspects of flower development in grasses. Sex Plant Reprod 24:247–282
Coen ES, Meyerowitz EM (1991) The war of the whorls: genetic interactions controlling flower development. Nature 353:31–37
Corbesier L, Coupland G (2006) The quest for florigen: a review of recent progress. J Exp Bot 57:3395–3403
Dinh TT, Girke T, Liu X, Yant L, Schmid M, Chen X (2012) The floral homeotic protein APETALA 2 recognizes and acts through an AT-rich sequence element. Development 139:1978–1986
Ditta G, Pinyopich A, Robles P, Pelaz S, Yanofsky MF (2004) The SEP4 gene of Arabidopsis thaliana functions in floral organ and meristem identity. Curr Biol 14:1935–1940
Endress PK (1996) Diversity and evolutionary biology of tropical flowers. Cambridge University Press, Cambridge
Google Scholar
Floyd SK, Bowman JL (2007) The ancestral developmental tool-kit of land plants. Int J Plant Sci 168:1–35
Article CAS Google Scholar
Friedman WE, Moore RC, Purgganan MD (2004) The evolution of plant development. Am J Bot 91:1726–1741
Article PubMed Google Scholar
Frohlich MW (2003) An evolutionary scenario for the origin of flowers. Nat Rev Genet 4:559–566
Hartmann U, Hohmann S, Nettesheim K, Wisman E, Saedler H, Huijser P (2000) Molecular cloning of SUP , a negative regulator of the floral meristem in Arabidopsis . Plant J 21:351–360
Hayama R, Yokoi S, Tamaki S, Yano M, Shimamoto K (2003) Adaptation of photoperiodic control pathways produces short-day flowering in rice. Nature 422:719–722
Huang T, Bohlenius H, Eriksson S, Parcy F, Nilsson O (2005) The mRNA of Arabidopsis gene FT moves from leaf to shoot apex and induces flowering. Science 309:1694–1696
Kaufmann K, Pajoro A, Angenet GC (2010) Regulation of transcription in plants: mechanism controlling developmental switches. Nat Rev Genet 11:830–842
Kidner CA, Martienssen RA (2005) The role of ARGONAUTE1 ( AGO1 ) in meristem formation and identity. Dev Biol 280:504–517
Kobayashi Y, Weigel D (2007) Move on up, it’s time for change: mobile signals controlling photoperiod-dependent flowering. Genes Dev 21:2371–2384
Krishnamurthy KV (2015) Growth and development in plants. Scientific Publishers, Jodhpur
Laux T (2003) The stem cell concept in plants: a matter of debate. Cell 113:281–283
Lenhard M, Bohnert A, Jurgens G, Laux T (2001) Termination of stem cell maintenance in Arabidopsis floral meristems by interactions between WUSCHEL and AGAMOUS . Cell 105:805–814
Li D, Liu C, Shen L, Wu Y, Chen H, Robertson M, Helliwell CA, Ito T, Meyerowitz E, Yu H (2008) A repressor complex governs the integration of flowering signals in Arabidopsis . Dev Cell 15:110–120
Lifschitz E, Eshed Y (2006) Universal florigenic signals triggered by FT homologues regulate growth and flowering cycles in perennial day-neutral tomato. J Exp Bot 57:3405–3414
Lohman JU, Hong RL, Hobe H, Busch MA, Parcy F, Simon R, Weigel DA (2001) Molecular link between stem cell regulation and floral patterning in Arabidopsis . Cell 105:793–803
Lohmann JU, Weigel D (2002) Building beauty: the genetic control of floral patterning. Dev Cell 2:135–142
Ma H (1997) To be, not to be, a flower–control of floral meristem identity. Trends Genet 14:26–32
Maizel A, Busch MA, Tanahashi T, Perkovic J, Kato M, Hasebe M, Weigel D (2005) The floral regulator LEAFY evolves by substitutions in the DNA binding domain. Science 308:260–263
Mondragon-Palomino M, Theissen G (2011) Conserved differential expression of paralogous Deficiens - and Globosa -like MADS-box genes in the flowers of Orchidaceae refining the ‘orchid code’. Plant J 66:1008–1018
Nougarède A (1967) Experimental cytology of the shoot apical cells during vegetative growth and flowering. Int Rev Cytol 21:203–351
Ó’Maoiléidigh DS, Graciet E, Wellmer F (2014) Gene networks controlling Arabidopsis thaliana flower development. New Phytol 201:16–30
Pelaz S, Ditta GS, Baumann E, Wisman E, Yanofsky MF (2000) B and C floral organ identity functions require SEPALLATA MADS-box genes. Nature 465:200–203
Posé D, Yant L, Schmid M (2012) The end of innocence: flowering networks explode in complexity. Curr Opin Plant Biol 15:45–50
Putterill J, Laurie R, Macknight R (2004) It’s time to flower: the genetic control of flowering time. Bioessays 26:363–373
Raghavan V (2000) Developmental biology of flowering plants. Springer, New York
Book Google Scholar
Riechmann JL, Wellmer F (2014) Flower development methods and protocols. Humana Press/Springer, New York
Salisbury F, Ross C (2005) Plant physiology. Easton Press, Norwalk
Salomé PA, Bomblies K, Laitinen RAE, Yant L, Mott R, Weigel D (2011) Genetic architecture of flowering-time variation in Arabidopsis thaliana . Genetics 188:421–433
Article PubMed Central PubMed Google Scholar
Soltis PS, Endress PK, Chase MW (2005) Phylogeny and evolution of angiosperms. Sinauer, Sunderland
Srikanth A, Schmid M (2011) Regulation of flowering time: all roads lead to Rome. Cell Mol Biol Life Sci 68:2013–2037
Sung S, Amasino RM (2004) Vernalization and epigenetics: how plants remember winter. Curr Opin Plant Biol 7:4–10
Swamy BGL, Krishnamurthy KV (1980) From flower to fruit-embryology of angiosperms. Tata McGraw-Hill, New Delhi
Theissen G, Melzer R (2007) Molecular mechanisms underlying origin and diversification of the angiosperm flower. Ann Bot 100:603–619
Theissen G, Saedler H (2001) Plant biology floral quartets. Nature 409:469–471
Theissen G, Becker A, Winter KU, Munster T, Kirchner C, Saedler H (2002) How the land plants learned their floral ABCs: the role of MADS box genes in the evolutionary origin of flowers. In: Cronk QC, Bateman RM, Hawkins JM (eds) Developmental genetics and plant evolution. Taylor & Francis, London
Valverde P, Mouradov A, Soppe W, Ravenscroft D, Samach A, Coupland G (2004) Photoreceptor regulator of CONSTANS protein in photoperiodic flowering. Science 303:1003–1006
Vijayaraghavan U (2001) How plants pattern flowers: lessons from molecular genetic studies of flowering in Arabidopsis thaliana , a model plant. Curr Sci 80:233–243
Vijayaraghavan U, Prasad K, Meyerowitz E (2005) Specification and maintenance of the floral meristem: interactions between positively-acting promoters of flowering and negative regulators. Curr Sci 89:1835–1844
Wagner D (2003) Chromatin regulation of plant development. Curr Opin Plant Biol 6:20–28
Wellmer F, Riechmann JL (2010) Gene networks controlling the initiation of flower development. Trends Genet 26:519–527
Wigge PA, Kim MC, Jaeger KE, Busch W, Schmidt M, Lohmann JU, Weigel D (2005) Integration of spatial and temporal information during floral induction in Arabidopsis . Science 309:1056–1059
Wollmann H, Mica E, Todesco M, Long JA, Weigel D (2010) On reconciling the interactions between APETALA2 , miR172 and AGAMOUS with the ABC model of flower development. Development 137:3633–3642
Wuest SE, Ó’Maoiléidigh DS, Rae I, Kwasniewska K, Raganelli A, Hanczaryk K, Lohan AJ, Loftus B, Graciet E, Wellmer F (2012) Molecular basic for the specification of floral organs by APETALA3 and PISTILLATA . Proc Natl Acad Sci U S A 109:13452–13457
Yamaguchi N, Wu MF, Winter CM, Berns MC, Nole-Wilson S, Yamaguchi A, Coupland G, Krizek BA, Wagner D (2013) A molecular framework for auxin-mediated initiation of flower primordia. Dev Cell 24:271–282
Yoshida H, Nagato Y (2011) Flower development in rice. J Exp Bot 62:4719–4730
Yu H, Ito T, Wellmer F, Meyerowitz EM (2004) Repression of AGAMOUS - LIKE24 is a crucial step in promoting flower development. Nature Genet 36:1557–1561
Zahn LM, Leebens-Mack J, de Pamphlis CW, Ma H, Theissen G (2005) To B or not to B a flower: the role of DEFICIENS and GLOBOSA orthologs in the evolution of the angiosperms. J Hered 96:225–240
Zeevaart JAD (1976) Physiology of flower formation. Annu Rev Plant Physiol 27:321–348
Zimmermann W (1952) Main results of the telome theory. Paleobotanist 1 (Birbal Sahni Memorial Volume): 456–470
Download references
Author information
Authors and affiliations.
Center for Pharmaceutics, Pharmacognosy and Pharmacology, School of Life Sciences, Institute of Trans-Disciplinary Health Science and Technology (IHST), Bangalore, Karnataka, India
K. V. Krishnamurthy
Sri Biotech Laboratories India Limited, Hyderabad, Telangana, India
Bir Bahadur
You can also search for this author in PubMed Google Scholar
Corresponding author
Correspondence to K. V. Krishnamurthy .
Editor information
Editors and affiliations.
Department of Genetics, University of Delhi, New Delhi, India
Manchikatla Venkat Rajam
Division of Biotechnology, Indian Institute of Horticultural Research (IIHR), Bangalore, Karnataka, India
Leela Sahijram
K.V. Krishnamurthy
Rights and permissions
Reprints and permissions
Copyright information
© 2015 Springer India
About this chapter
Krishnamurthy, K.V., Bahadur, B. (2015). Genetics of Flower Development. In: Bahadur, B., Venkat Rajam, M., Sahijram, L., Krishnamurthy, K. (eds) Plant Biology and Biotechnology. Springer, New Delhi. https://doi.org/10.1007/978-81-322-2286-6_16
Download citation
DOI : https://doi.org/10.1007/978-81-322-2286-6_16
Publisher Name : Springer, New Delhi
Print ISBN : 978-81-322-2285-9
Online ISBN : 978-81-322-2286-6
eBook Packages : Biomedical and Life Sciences Biomedical and Life Sciences (R0)
Share this chapter
Anyone you share the following link with will be able to read this content:
Sorry, a shareable link is not currently available for this article.
Provided by the Springer Nature SharedIt content-sharing initiative
- Publish with us
Policies and ethics
- Find a journal
- Track your research
- Advanced search
- Peer review
- Record : found
- Abstract : found
- Article : not found
The ABC model of flower development: then and now.
Read this article at.
- open (via free pdf)
- oa repository (via OAI-PMH doi match)

- Review article
- Invite someone to review
Abstract
Author and article information , comment on this article.
- Help & FAQ
The ABC model of flower development: then and now
- School of Biological Sciences
Research output : Contribution to journal › Comment / Debate › Research › peer-review
Access to Document
- 10.1242/dev.083972
Other files and links
- RM sElocation
T1 - The ABC model of flower development: then and now
AU - Bowman, John Lincoln
AU - Smyth, David Robert
AU - Meyerowitz, Elliot M
N2 - In 1991, we published a paper in Development that proposed the ABC model of flower development, an early contribution to the genetic analysis of development in plants. In this, we used a series of homeotic mutants, and double and triple mutants, to establish a predictive model of organ specification in developing flowers. This model has served as the basis for much subsequent work, especially towards understanding seed plant evolution. Here, we discuss several aspects of this story, that could be a much longer one. One surprising conclusion is that materials and methods that might have led to similar work, and to the same model, were available 100 years before our experiments, belying the belief that progress in biology necessarily comes from improvements in methods, rather than in concepts.
AB - In 1991, we published a paper in Development that proposed the ABC model of flower development, an early contribution to the genetic analysis of development in plants. In this, we used a series of homeotic mutants, and double and triple mutants, to establish a predictive model of organ specification in developing flowers. This model has served as the basis for much subsequent work, especially towards understanding seed plant evolution. Here, we discuss several aspects of this story, that could be a much longer one. One surprising conclusion is that materials and methods that might have led to similar work, and to the same model, were available 100 years before our experiments, belying the belief that progress in biology necessarily comes from improvements in methods, rather than in concepts.
UR - http://dev.biologists.org/content/139/22/4095.full.pdf+html
U2 - 10.1242/dev.083972
DO - 10.1242/dev.083972
M3 - Comment / Debate
SN - 1011-6370
JO - Development
JF - Development
MOSCOW - RUSSIA
Ewf b.v east west forwarding.
Edelveis, Right Entrance, 2nd Floor Davidkovskaja, 121352 Moscow, Russia
- Phone: +7 495 938-99-66
- Mobile: +7 495-997-0977
- Fax: +7 495 938-99-67
- email: [email protected]
- web: www.eastwestforwarding.com
Company Profile
- LIST WITH US
To: EWF B.V EAST WEST FORWARDING
Enter the security code:
+7 495 938-99-67
+7 495-997-0977
+7 495 938-99-66
Directory of Freight Forwarders, Cargo Agents, Shipping Companies, Air, Ocean, Land, Logistics and Transportation Brokers

- Yekaterinburg
- Novosibirsk
- Vladivostok

- Tours to Russia
- Practicalities
- Russia in Lists
Rusmania • Deep into Russia
Out of the Centre
Savvino-storozhevsky monastery and museum.

Zvenigorod's most famous sight is the Savvino-Storozhevsky Monastery, which was founded in 1398 by the monk Savva from the Troitse-Sergieva Lavra, at the invitation and with the support of Prince Yury Dmitrievich of Zvenigorod. Savva was later canonised as St Sabbas (Savva) of Storozhev. The monastery late flourished under the reign of Tsar Alexis, who chose the monastery as his family church and often went on pilgrimage there and made lots of donations to it. Most of the monastery’s buildings date from this time. The monastery is heavily fortified with thick walls and six towers, the most impressive of which is the Krasny Tower which also serves as the eastern entrance. The monastery was closed in 1918 and only reopened in 1995. In 1998 Patriarch Alexius II took part in a service to return the relics of St Sabbas to the monastery. Today the monastery has the status of a stauropegic monastery, which is second in status to a lavra. In addition to being a working monastery, it also holds the Zvenigorod Historical, Architectural and Art Museum.
Belfry and Neighbouring Churches

Located near the main entrance is the monastery's belfry which is perhaps the calling card of the monastery due to its uniqueness. It was built in the 1650s and the St Sergius of Radonezh’s Church was opened on the middle tier in the mid-17th century, although it was originally dedicated to the Trinity. The belfry's 35-tonne Great Bladgovestny Bell fell in 1941 and was only restored and returned in 2003. Attached to the belfry is a large refectory and the Transfiguration Church, both of which were built on the orders of Tsar Alexis in the 1650s.

To the left of the belfry is another, smaller, refectory which is attached to the Trinity Gate-Church, which was also constructed in the 1650s on the orders of Tsar Alexis who made it his own family church. The church is elaborately decorated with colourful trims and underneath the archway is a beautiful 19th century fresco.
Nativity of Virgin Mary Cathedral

The Nativity of Virgin Mary Cathedral is the oldest building in the monastery and among the oldest buildings in the Moscow Region. It was built between 1404 and 1405 during the lifetime of St Sabbas and using the funds of Prince Yury of Zvenigorod. The white-stone cathedral is a standard four-pillar design with a single golden dome. After the death of St Sabbas he was interred in the cathedral and a new altar dedicated to him was added.

Under the reign of Tsar Alexis the cathedral was decorated with frescoes by Stepan Ryazanets, some of which remain today. Tsar Alexis also presented the cathedral with a five-tier iconostasis, the top row of icons have been preserved.
Tsaritsa's Chambers

The Nativity of Virgin Mary Cathedral is located between the Tsaritsa's Chambers of the left and the Palace of Tsar Alexis on the right. The Tsaritsa's Chambers were built in the mid-17th century for the wife of Tsar Alexey - Tsaritsa Maria Ilinichna Miloskavskaya. The design of the building is influenced by the ancient Russian architectural style. Is prettier than the Tsar's chambers opposite, being red in colour with elaborately decorated window frames and entrance.

At present the Tsaritsa's Chambers houses the Zvenigorod Historical, Architectural and Art Museum. Among its displays is an accurate recreation of the interior of a noble lady's chambers including furniture, decorations and a decorated tiled oven, and an exhibition on the history of Zvenigorod and the monastery.
Palace of Tsar Alexis

The Palace of Tsar Alexis was built in the 1650s and is now one of the best surviving examples of non-religious architecture of that era. It was built especially for Tsar Alexis who often visited the monastery on religious pilgrimages. Its most striking feature is its pretty row of nine chimney spouts which resemble towers.

Plan your next trip to Russia
Ready-to-book tours.
Your holiday in Russia starts here. Choose and book your tour to Russia.
REQUEST A CUSTOMISED TRIP
Looking for something unique? Create the trip of your dreams with the help of our experts.

IMAGES
VIDEO
COMMENTS
ABC model of flower development guided by three groups of homeotic genes.. The ABC model of flower development is a scientific model of the process by which flowering plants produce a pattern of gene expression in meristems that leads to the appearance of an organ oriented towards sexual reproduction, a flower.There are three physiological developments that must occur in order for this to take ...
The ABC model of flower development in angiosperm demonstrates the presence of three classes of genes that regulate the development of floral organs. The genes are referred to as class A genes, class B genes and class C gene. These genes and the interaction between them induce the development of floral organs.
In 1991, we published a paper in Development that proposed the ABC model of flower development, an early contribution to the genetic analysis of development in plants. In this, we used a series of homeotic mutants, and double and triple mutants, to establish a predictive model of organ specification in developing flowers. This model has served as the basis for much subsequent work, especially ...
Wild type Arabidopsis flower (A), color coded in (B) to demarcate the sepals (red), petals (purple), stamens (green) and carpels (yellow). (C) A cartoon version of an Arabidopsis flower, with the domains of ABC gene function shown below. The function of A alone specifies sepal identity (red) in the first whorl, while a combination of A (red) + B (blue) function specifies petal identity (purple ...
Early descriptions of flower variants. A) Single- (left) and double-flowered (right) wallflowers, Erysimum cheiri (Gerard et al. 1597). B) An apple mutant in which the petals are transformed to another whorl of sepals and the stamens are transformed into carpels. This tree was used for cross-fertilization, where "the ladies and young girls of Saint-Valery compete to 'make their apple ...
According to the ABC model of flower development, the four types of floral organs in a typical flower, ... but also help test the hypothesis derived from comparative investigations. Second, the ...
The ABC model for flower development describes typical higher EUDICOT flowers that consist of sepals, ... A recent QTL analysis of A. thaliana leaf and floral-organ size supports this hypothesis: ...
Introduction. Specification of organ identity during flower development is explained by the ABC model. Since its inception in 1988 (Haughn and Somerville, 1988), the model was modified several times to adapt to experimental findings and conceptual progress (Coen and Meyerowitz, 1991; Theißen et al., 2000; Theißen, 2001; Litt, 2007; Causier et al., 2010; Wellmer et al., 2014).
In Chapter 9 we discussed floral organ development, and the functions of a set of transcription factors in activating the structural genes necessary for correct organ development in the correct position. The majority of these ABC genes encode MADS box transcription factors, a very ancient family of DNA-binding proteins. By tracing the evolutionary history of this gene family we can investigate ...
4095. Summary. In 1991, we published a paper in Development that proposed. the ABC model of flower development, an early contribution to. the genetic analysis of development in plants. In this, we ...
The ABC model. Wild type Arabidopsis flower (A), color coded in (B) to demarcate the sepals (red), petals (purple), stamens (green) and carpels (yellow). (C) A cartoon version of an Arabidopsis flower, with the domains of ABC gene function shown below. The function of A alone specifies sepal identity (red) in the first whorl, while a ...
Figure 30.12.1 30.12. 1: ABC model of flower development: Class A genes (blue) affect sepals and petals, class B genes (yellow) affect petals and stamens, class C genes (red) affect stamens and carpels. Most genes central in this model belong to the MADS-box genes and are transcription factors that regulate the expression of the genes specific ...
Flower development: ... In the ABC model, a flower is composed of four concentric whorls bearing different kind of floral organs—sepals, petals, stamens, and carpels—sequentially arranged from the outer to the inner part of the flower. ... This strongly supports the hypothesis of an ancient divergence of clades I and II, where clade I might ...
Development. the ABC model of flower development, an early contribution to the genetic analysis of development in plants. In this, we used a series of homeotic mutants, and double and triple ...
The paper introduces the classical ABC model of floral development and thereafter ABCD, ABCDE and quartet models, and presents achievements in the studies on floral ...
Another hypothesis for the origin of flowers has suggested a homeotic transformation of reproductive organs to either male or female ... The ABC model of flower development: then and now. Development 139:4095-4098. Article CAS PubMed Google Scholar Chen X (2004) A microRNA as a translational repressor of APETALA2 in Arabidopsis flower ...
In 1991, we published a paper in Development that proposed the ABC model of flower development, an early contribution to the genetic analysis of development in plants. In this, we used a series of homeotic mutants, and double and triple mutants, to establish a predictive model of organ specification in developing flowers. This model has served as the basis for much subsequent work, especially ...
In further support of Goethe's hypothesis, ... Comparative genomics combined with new models for research on flower development will undoubtedly be necessary to make significant progress in this area, but there are already impressive case studies to understand the genetic basis of floral shape variation. ... The ABC model of flower development ...
Map of the Moscow Oblast. The Joseph-Volokolamsk Monastery in Volokolamsk. Flag Coat of arms. Moscow Oblast (Russian: Моско́вская о́бласть, Moskovskaya oblast) is a federal subject of Russia.It is located in western Russia, and it completely surrounds Moscow.The oblast has no capital, and oblast officials reside in Moscow or in other cities within the oblast.
Dmitriy V. Mikheev, Karina A. Telyants, Elena N. Klochkova, Olga V. Ledneva; Affiliations Dmitriy V. Mikheev
Abstract. In 1991, we published a paper in Development that proposed the ABC model of flower development, an early contribution to the genetic analysis of development in plants. In this, we used a series of homeotic mutants, and double and triple mutants, to establish a predictive model of organ specification in developing flowers.
EWF B.V EAST WEST FORWARDING. Edelveis, Right Entrance, 2nd Floor Davidkovskaja, 121352 Moscow, Russia. Phone: +7 495 938-99-66; Mobile: +7 495-997-0977
Zvenigorod's most famous sight is the Savvino-Storozhevsky Monastery, which was founded in 1398 by the monk Savva from the Troitse-Sergieva Lavra, at the invitation and with the support of Prince Yury Dmitrievich of Zvenigorod. Savva was later canonised as St Sabbas (Savva) of Storozhev. The monastery late flourished under the reign of Tsar ...