
An official website of the United States government
The .gov means it’s official. Federal government websites often end in .gov or .mil. Before sharing sensitive information, make sure you’re on a federal government site.
The site is secure. The https:// ensures that you are connecting to the official website and that any information you provide is encrypted and transmitted securely.
- Publications
- Account settings
Preview improvements coming to the PMC website in October 2024. Learn More or Try it out now .
- Advanced Search
- Journal List
- Physiol Rev


About Sleep's Role in Memory
Over more than a century of research has established the fact that sleep benefits the retention of memory. In this review we aim to comprehensively cover the field of “sleep and memory” research by providing a historical perspective on concepts and a discussion of more recent key findings. Whereas initial theories posed a passive role for sleep enhancing memories by protecting them from interfering stimuli, current theories highlight an active role for sleep in which memories undergo a process of system consolidation during sleep. Whereas older research concentrated on the role of rapid-eye-movement (REM) sleep, recent work has revealed the importance of slow-wave sleep (SWS) for memory consolidation and also enlightened some of the underlying electrophysiological, neurochemical, and genetic mechanisms, as well as developmental aspects in these processes. Specifically, newer findings characterize sleep as a brain state optimizing memory consolidation, in opposition to the waking brain being optimized for encoding of memories. Consolidation originates from reactivation of recently encoded neuronal memory representations, which occur during SWS and transform respective representations for integration into long-term memory. Ensuing REM sleep may stabilize transformed memories. While elaborated with respect to hippocampus-dependent memories, the concept of an active redistribution of memory representations from networks serving as temporary store into long-term stores might hold also for non-hippocampus-dependent memory, and even for nonneuronal, i.e., immunological memories, giving rise to the idea that the offline consolidation of memory during sleep represents a principle of long-term memory formation established in quite different physiological systems.
I. INTRODUCTION
The capability to form memory is critical to the strategic adaptation of an organism to changing environmental demands. Observations indicating that sleep benefits memory date back to the beginning of experimental memory research, and since then have been fitted with quite different concepts. This review targets this field of “sleep and memory” research, which has experienced a unique renaissance during the last three decades. Although we have aimed at comprehensively covering the field, we might have missed out or overlooked some aspects, owing to the vast progress achieved in the last years. Before we begin, we will briefly introduce the core concepts of sleep and memory, respectively.
Sleep is defined as a natural and reversible state of reduced responsiveness to external stimuli and relative inactivity, accompanied by a loss of consciousness. Sleep occurs in regular intervals and is homeostatically regulated, i.e., a loss or delay of sleep results in subsequently prolonged sleep ( 113 ). Sleep deprivation and sleep disruptions cause severe cognitive and emotional problems ( 142 , 634 , 1243 ), and animals deprived of sleep for several weeks show temperature and weight dysregulation and ultimately die of infections and tissue lesions ( 973 ). Sleep probably occurs in all vertebrates, including birds, fishes, and reptiles, and sleeplike states are similarly observed in invertebrates like flies, bees, and cockroaches ( 209 ).
Sleep in mammals consists of two core sleep stages: slow-wave sleep (SWS) and rapid-eye-movement (REM) sleep, which alternate in a cyclic manner ( FIGURE 1 A ). In human nocturnal sleep, SWS is predominant during the early part and decreases in intensity and duration across the sleep period, whereas REM sleep becomes more intense and extensive towards the end of the sleep period. SWS is hallmarked by slow high-amplitude EEG oscillations (slow wave activity, SWA), whereas REM sleep (also termed paradoxical sleep) is characterized by wakelike fast and low-amplitude oscillatory brain activity. In addition, REM sleep is characterized by phasic REMs and by muscle atonia. Almost 50% of sleep in adult humans is marked by a lighter form of non-REM sleep (stage “N2”) that is characterized by the occurrence of distinct (waxing and waning) sleep spindles ( FIGURE 1 B ) and K-complexes in the EEG, but minor SWA. Sleep stage N2 is not discriminated from SWS in rodents.

Typical human sleep profile and sleep-related signals. A : sleep is characterized by the cyclic occurrence of rapid-eye-movement (REM) sleep and non-REM sleep. Non-REM sleep includes slow-wave sleep (SWS) corresponding to N3, and lighter sleep stages N1 and N2 ( 591 ). According to an earlier classification system by Rechtschaffen and Kales ( 974 ), SWS was divided into stage 3 and stage 4 sleep. The first part of the night (early sleep) is dominated by SWS, whereas REM sleep prevails during the second half (late sleep). B : the most prominent electrical field potential oscillations during SWS are the neocortical slow oscillations (∼0.8 Hz), thalamocortical spindles (waxing and waning activity between 10–15 Hz), and the hippocampal sharp wave-ripples (SW-R), i.e., fast depolarizing waves that are generated in CA3 and are superimposed by high-frequency (100–300 Hz) ripple oscillation. REM sleep, in animals, is characterized by ponto-geniculo-occipital (PGO) waves, which are associated with intense bursts of synchronized activity propagating from the pontine brain stem mainly to the lateral geniculate nucleus and visual cortex, and by hippocampal theta (4–8 Hz) activity. In humans, PGO and theta activity are less readily identified. C : sleep is accompanied by a dramatic change in activity levels of different neurotransmitters and neuromodulators. Compared with waking, cholinergic activity reaches a minimum during SWS, whereas levels during REM sleep are similar or even higher than those during waking. A similar pattern is observed for the stress hormone cortisol. Aminergic activity is high during waking, intermediate during SWS, and minimal during REM sleep. [Modified from Diekelmann and Born ( 293 ).]
Is sleep essential? From an evolutionary perspective, reduced responsiveness to potentially threatening stimuli during sleep represents a significant danger to survival. The fact that almost all animals sleep strongly argues in favor of an adaptive role of sleep in increasing the overall fitness of an organism, although its exact functions are still a matter of debate ( 407 , 1077 ). Sleep has been proposed as serving an energy-saving function ( 82 , 1311 ), the restoration of energy resources and the repairing of cell tissue ( 875 ), thermoregulation ( 973 ), metabolic regulation ( 651 , 1229 ), and adaptive immune functions ( 695 ). However, these functions could be likewise achieved in a state of quiet wakefulness and would not explain the loss of consciousness and responsiveness to external threats during sleep. These prominent features of sleep strongly speak for the notion that sleep is mainly “for the brain” ( 553 , 625 ). Here, different functions have been proposed, ranging from detoxication of the brain from free radicals ( 594 , 978 ), glycogen replacement ( 1041 ) to an involvement of sleep in memory and synaptic plasticity ( 293 , 1204 ). In this review we discuss this latter function, i.e., the critical role sleep serves in the formation of memory.
1. Memory processes
To form and retrieve memories is a fundamental ability of any living organism, enabling it to adapt its behavior to the demands of an ever-changing environment, and allowing it to appropriately select and improve the behaviors of a given repertoire. Memory functions comprise three major subprocesses, i.e., encoding, consolidation, and retrieval. During encoding, the perception of a stimulus results in the formation of a new memory trace, which is initially highly susceptible to disturbing influences and decay, i.e., forgetting. During consolidation, the labile memory trace is gradually stabilized possibly involving multiple waves of short and long-term consolidation processes ( 803 ), which serve to strengthen and integrate the memory into preexisting knowledge networks. During retrieval, the stored memory is accessed and recalled. This review discusses sleep's critical role in the consolidation of memory. We assume that whereas the waking brain is optimized for the acute processing of external stimuli that involves the encoding of new information and memory retrieval, the sleeping brain provides optimal conditions for consolidation processes that integrate newly encoded memory into a long-term store. Encoding and consolidation might be mutually exclusive processes inasmuch they draw on overlapping neuronal resources. Thus sleep as a state of greatly reduced external information processing represents an optimal time window for consolidating memories.
The so-called consolidation account of memory processing was first proposed by Müller and Pilzecker ( 840 ) who, based on studies of retroactive interference between learning lists of syllables, concluded: “After all this, there is no alternative but to assume that after reading a list of syllables certain physiological processes, which serve to strengthen the associations induced during reading of that list, continue with decreasing intensity for a period of time.” (p. 196 in Ref. 840 , cited based on Ref. 709 ). The consolidation hypothesis is now widely accepted based on numerous studies showing that psychological, pharmacological, and electrophysiological manipulations, such as interference learning, the administration of norepinephrine and protein synthesis inhibitors or electroconvulsive shocks, can effectively impair or enhance memory, when administered after encoding (e.g., Refs. 803 , 1332 ). Importantly, these manipulations are time dependent and have strongest effects when applied immediately after learning (for reviews, see Refs. 194 , 805 ). The consolidation possibly involves multiple waves of stabilizing processes, which exhibit different time courses and depend on different underlying processes of neuronal plasticity. Recent evidence suggests that memory traces are not consolidated once but, upon their reactivation by a reminder or active retrieval, undergo a period of reconsolidation to persist for the long term ( 844 ).
At the neuronal level, memory formation is thought to be based on the change in the strength of synaptic connections in the network representing the memory. Encoding induces synaptic long-term potentiation (LTP) or long-term depression (LTD) as major forms of learning-induced synaptic plasticity ( 222 , 527 , 616 , 629 , 1209 ). Activity reverberating in the neuronal representation following encoding is thought to promote two kinds of consolidation processes, termed “synaptic consolidation” and “systems consolidation” ( 330 ). Synaptic consolidation leads to the remodeling of the synapses and spines of the neurons contributing to a memory representation, eventually producing enduring changes in the efficacy of the participating synapses (e.g., Refs. 555 , 616 , 977 ). System consolidation builds on synaptic consolidation and refers to processes in which reverberating activity in newly encoded representations stimulate a redistribution of the neuronal representations to other neuronal circuitries for long-term storage ( 418 ).
2. Memory systems
In neuropsychology, declarative and nondeclarative memory systems are distinguished depending on the critical involvement of medial temporal lobe regions, particularly of the hippocampus, in the acquisition of memory ( 1134 ). Declarative memory encompasses 1 ) episodic memories for events that are embedded in a spatiotemporal context (including autobiographical memories) and 2 ) semantic memories for facts that are stored independently of contextual knowledge ( 1218 ). Declarative memories can be encoded intentionally or unintentionally, but are typically explicitly (i.e., with awareness) accessible by active recall attempts. Episodic memories are learned very quickly, i.e., in one trial, but are also subject to fast forgetting ( 1332 ). Semantic memories can be regarded as a result of the repeated encoding or activation of overlapping episodic memories ( 1327 ). Integrity of hippocampal circuitry is a prerequisite for retaining an episode as well as spatial and temporal context information in memory for more than 15 min ( 231 , 418 ).
In contrast to declarative memories, nondeclarative memories can be acquired without involvement of medial temporal lobe structures ( 1134 ). Nondeclarative memory encompasses quite different memory systems that rely on different areas of the brain. It includes procedural memories for motor skills (motor areas, striatum, cerebellum) and perceptual skills (sensory cortices), certain forms of conditioning and implicit learning (priming), etc. Nondeclarative memories can be implicitly (i.e., without awareness) acquired and recalled, and learning is slow, usually requiring multiple training trials. It is of note that experimentally disentangling nondeclarative from declarative memory processing is often complicated by the fact that these memory systems interact during acquisition of new knowledge in the healthy brain. Thus acquisition of skills like language learning and finger sequence tapping, especially at the initial stages, incorporates declarative in addition to procedural components ( 910 ).
3. The standard two-stage memory system
Why does the consolidation of memory have to take place during sleep? The hypotheses that sleep serves memory consolidation is conceptually rooted in the standard two-stage memory system which is currently the most influential model of human memory, and has been developed as a solution to several key problems arising from simple associative network models of memory ( 175 , 780 , 800 ). The foremost of these problems is that although simple association networks are in fact able to store information very rapidly, as is the case in the declarative memory system, the uptake of new conflicting information has a strong tendency to erase the older memories, thus inducing so-called “catastrophic interference” ( 1005 ). The critical question is how the neuronal network can learn new patterns without simultaneously forgetting older memories, an issue that has also been referred to as the “stability-plasticity dilemma” (e.g., see Ref. 3 ). In addition, unstructured recurrent networks have been demonstrated to face essential capacity constraints ( 723 ). The two-stage memory formation mechanism first proposed by Marr ( 780 ) offers a solution to these problems. It assumes that memories are initially encoded into a fast learning store (i.e., the hippocampus in the declarative memory system) and then gradually transferred to a slow learning store for long-term storage (i.e., the neocortex). The fast learning store ensures quick and efficient encoding of memories, even in one attempt (one-trial learning). Yet, these representations are unstable and vulnerable to (retroactive) interference by newly encoded information. Over time, the information is gradually integrated in the slowly learning long-term store without overwriting older, more remote memories. It is assumed that by the repeated reactivation of the new memories during off-line periods like sleep, the slowly learning long-term store is trained and the new memories are gradually strengthened and adapted to preexisting long-term memories. The transformation new memory representations undergo in this system consolidation process comprises also the extraction of invariants and the development of prototypes and schemas, as the core of the newly learned information is reactivated more frequently than divergent details ( 734 , 800 , 1239 ). For the declarative memory system, the two-stage model has received strong support from lesion studies, indicating that lesions of the hippocampus abolish the ability to acquire new declarative memory and simultaneously produce a temporally graded retrograde amnesia where older memories remain intact ( 231 , 418 ). The time interval for a memory to reach a state of hippocampus-independent retrieval can vary from one day to several months or years, depending on the acquired information and the schemas preexisting in long-term memory ( 1210 , 1308 ). The standard two-stage model of memory has been also successfully applied to nondeclarative kinds of memory, like procedural memory ( 668 ), suggesting that the offline reactivation of recent memories and their redistribution from a fast encoding temporary to a slowly learning permanent store could be a general feature of long-term memory formation.
II. OVERVIEW OF APPROACHES AND CONCEPTS
In this section, we review evidence from behavioral studies in support of the notion that sleep benefits memory consolidation. Key experiments for the different theoretical accounts and concepts will be described in more or less chronological order, thus complementing previous reviews of studies on sleep and memory ( 23 , 49 , 99 , 116 , 121 , 125 , 199 , 200 , 226 , 246 , 251 , 293 , 297 , 319 , 348 , 377 , 379 , 411 , 475 , 524 , 537 , 538 , 734 , 765 , 782 , 807 , 883 , 894 , 910 , 915 , 928 , 957 , 958 , 967 , 984 , 988 , 1001 , 1025 , 1092 , 1094 , 1096 , 1098 , 1149 , 1151 , 1152 , 1156 , 1202 , 1242 , 1279 – 1281 , 1283 , 1289 – 1291 , 1305 , 1321 ).
A. Sleep Acts by Passively Protecting Memory From Retroactive Interference
In 1885, Ebbinghaus, the father of experimental memory research, published a series of studies, on himself, about the forgetting of lists of nonsense-word pairs that established the well-known “forgetting curve” indicating that forgetting occurs rapidly in the first hours after learning and levels out after several days ( 339 ). He noticed already in this work that forgetting is reduced when sleep occurred in the retention interval, a phenomenon similarly observed in follow-up studies examining the forgetting curve (reviewed in Ref. 1242 ). Others reported that depriving a participant of a night of sleep impaired his ability to remember ( 890 ). Rosa Heine ( 528 , 1046 ) was the first to show in a more systematic study (in 6 subjects) that learning in the evening before sleep resulted in less forgetting 24 h later than learning before a daytime retention interval of wakefulness. This work provided the first clues as to the importance of sleep for memory.
Memory research in the first half of the 20th century was preoccupied with the cause of forgetting. Two concepts were proposed, i.e., the “decay” account, assuming that memory traces decay over time resulting in time-dependent forgetting ( 1188 ), and the “interference” account, assuming that forgetting results from learning of new information which (retroactively) interferes and overwrites the old memory traces ( 806 ). In a classic study, Jenkins and Dallenbach ( 603 ) compared (in two participants, which were repeatedly examined every day and night over a period of almost 2 mo) the retention of nonsense syllables across 1-, 2-, 4-, and 8-h retention periods that were filled either with sleep or wakefulness ( FIGURE 2 ) . Sleep after learning reduced the amount of forgetting. Because the time retention interval was identical for the sleep and awake conditions, the authors concluded that “… results of our study as a whole indicate that forgetting is not so much a matter of the decay of old impressions and associations as it is a matter of interference, inhibition, or obliteration of the old by the new” (p. 612 in Ref. 603 ). Because sleep represents a time in which new encoding of external and, perhaps, also internal information is strongly reduced, the reduction of interference by sleep appears to be crucial. However, the findings by Jenkins and Dallenbach also pose a challenge to the interference theory, because learning of highly similar material did not occur during the waking periods in these studies. Interference is considered to depend on the similarity between learning and interference materials with stronger interference for highly similar tasks (see Ref. 632 for a review). Regardless of this issue, the findings were interpreted as evidence that any waking mental activity increases forgetting by a kind of nonspecific interference ( 1332 ).

Effects of sleep and wake intervals of different length after learning on memory for senseless syllables. Sleep after learning leads to superior recall of syllables after the 1-, 2-, 4-, and 8-h retention interval, compared with wake intervals of the same length. Two subjects (H. and Mc.) participated in this classic study by Jenkins and Dallenbach ( 603 ). For each data point, each participant completed 6–8 trials, with the different retention intervals performed in random order. The study took ∼2 mo during which the participants lived in the laboratory and were tested almost every day and night. Data are based on Table 3 in Reference 603 , as the original figure contains an erroneous exchange of data points at the 4-h wake retention interval. Values are means ± SE. ** P ≤ 0.01; *** P ≤ 0.001.
Many studies subsequently confirmed the positive effect of sleep on memory ( 63 , 79 , 80 , 248 , 341 – 343 , 350 , 408 , 478 , 479 , 592 , 745 , 853 , 855 , 1068 , 1131 , 1192 , 1241 ), examining also longer retention intervals of from 24 h up to 6 days ( 79 , 80 , 474 , 592 , 990 ). The underlying concept was that sleep acts as a “temporary shelter” that simply postpones the effect of interference and, thereby, passively maintains the memory traces (p. 717 in Ref. 348 ). However, the pure hypothesis that simply the amount of interference between learning and recall determines the degree of forgetting is critically challenged by the fact that effects of retroactive interference are time dependent and much stronger when occurring immediately after learning than at a later time, speaking in favor of a time-dependent process of consolidation after encoding that strengthens the original memory trace, rendering it less susceptible to interference with time ( 840 ).
A time dependency of the effects of sleep on memory formation is indicated by studies showing stronger effects for sleep occurring shortly after learning than for sleep at a later time ( 80 , 343 , 431 , 899 , 1172 ). For example, sleep occurring within 3 h after learning vocabulary was more beneficial than sleep delayed by more than 10 h ( 431 ). Furthermore, recall of word pairs after 24 h was better when sleep occurred immediately after learning than after a day of wakefulness ( 899 ). Importantly, because the time between learning and retrieval as well as the time spent sleeping was identical for the immediate versus delayed sleep conditions of these studies, the findings cannot be explained by interference reduction per se, but stress the importance of the timing of reduced interference with reference to the learning period. That sleep after learning actually benefits the consolidation of memories and strengthens memory traces against future interference was compellingly demonstrated by Ellenbogen and co-workers ( 346 , 347 ). In two studies, they revealed that the enhancing effect on word recall of sleep compared with wakefulness was strongly enhanced when the subjects had learned an interference list shortly before final recall testing. Further studies confirmed that a 90-min sleep period as well as 60-min naps, both containing mainly SWS, likewise protect memory against future interference ( 18 , 290 , 1069 ).
In fact, recent versions of the interference account on sleep-associated memory consolidation have integrated this issue, assuming that sleep provides a time of reduced interference on consolidation processes, which per se are considered to be time dependent ( 809 , 1332 ). Thus any treatment that reduces interfering influences on consolidation should be more effective the shorter it is applied after learning. Still, these interference accounts assume a passive “opportunistic” role of sleep in memory consolidation occurring regardless of whether the brain is asleep or awake ( 809 ). Yet, with the assumption that sleep generally reduces interference from encoding of external events, this theorizing is challenged by a great body of studies indicating a dependence of consolidation on the composition of sleep, with differential outcomes for sleep rich of REM sleep or SWS ( 63 , 343 , 408 , 930 , 931 , 1048 , 1340 ). Thus explaining the improving effect of sleep on memory retention solely on the basis of reduced interference appears to be untenable unless sleep stages are thought to differ in their degree of interference, e.g., owing to associated dreaming ( 408 ).
B. REM Sleep and Memory Consolidation
The hypothesis has been around for some time that REM sleep contributes to memory consolidation, stimulated in particular by the wakelike EEG activity during this sleep stage together with frequent reports of vivid dreams after awakening from REM sleep. Very consistent evidence for a role of REM sleep for memory was provided by studies in animals (for comprehensive reviews, see, e.g., Refs. 101 , 388 , 807 , 901 , 936 , 943 , 1092 , 1095 , 1098 , 1260 ). With the use of a variety of tasks including classic, aversive, and appetitive conditioning procedures, a large number of studies consistently revealed increases in REM sleep after learning in rats, mice, and cats ( 100 , 284 , 389 , 540 , 708 , 711 – 713 , 747 , 944 , 1102 , 1104 , 1110 , 1111 ). Rats living in enriched compared with impoverished environments likewise exhibited enhanced REM sleep, although increases in non-REM sleep were also observed ( 278 , 493 , 494 , 669 , 823 , 1086 , 1168 ). Increasing REM pharmacologically by administration of carbachol into the pontine reticular formation and of corticotrophin-like intermediate lobe peptide (CLIP) as well as a REM sleep rebound after prior REM deprivation, all improved memory for a Y-maze discrimination task when applied after learning of the task ( 1316 ). Deprivation of REM sleep (mostly without simultaneous sleep recording) appeared to primarily impair memory formation on complex tasks, like two-way shuttle box avoidance and complex mazes, which encompass a change in the animals regular repertoire ( 69 , 100 , 312 , 516 , 525 , 539 , 644 , 710 , 713 , 714 , 787 , 900 , 903 – 906 , 992 , 1021 , 1072 , 1111 , 1113 , 1238 , 1352 , 1353 ). In contrast, long-term memory for simpler tasks, like one-way active avoidance and simple mazes, were less consistently affected ( 15 , 249 , 386 , 390 , 495 , 558 , 611 , 644 , 821 , 872 , 902 , 907 – 909 , 1072 , 1091 , 1334 ).
REM sleep increases were mostly observed in the first hours after learning, partly reflecting the fact that recording was limited to these hours. With prolonged recording sessions, elevated periods of REM sleep occurred up to 4–6 days after learning, sometimes following a cyclic pattern ( 1111 , 1116 ). Here, REM sleep increases were typically most prominent during specific time periods and dependent on the task. In the Morris water maze task, it started more than 2 h after learning and persisted for 22 h ( 1099 ). In several other avoidance tasks, REM sleep increases were less persistent, emerged later (i.e., 9–12 h post-learning), and sometimes reemerged 17–20 h post-learning ( 1094 ). In appetitive learning tasks, REM sleep increases started after 4 h and persisted for 12 h ( 1104 ). The increases in REM sleep during the specific time periods predicted later memory recall and reliably separated between learners and nonlearners ( 1095 , 1110 ). Learning in these studies induced distinct and prolonged waves of REM increases possibly involved in memory formation. Based on these findings, Smith proposed the concept of “paradoxical sleep windows” (PSW) mediating memory formation ( 1092 , 1097 ). Indeed, selective deprivation of REM sleep during, but not outside of, identified PSWs impaired memory ( 719 , 1101 , 1105 , 1106 , 1113 , 1115 ). Inhibition of protein synthesis by anisomycin also impaired memory only when intraperitoneally injected during a PSW 9 h after learning a shuttle avoidance task ( 1108 ). Similar results were obtained when blocking muscarinic cholinergic receptors during a PSW by scopolamine ( 720 , 1108 ), pointing to a crucial involvement of protein synthesis as well as cholinergic activation in PSW-associated memory processes. Interestingly, blocking of NMDA receptors was most effective in impairing memory consolidation when administered after a PSW, suggesting that PSW-associated memory processing induces subsequent NMDA-dependent plasticity ( 1094 , 1099 ).
Quite a number of studies in this context have been criticized as they employed the “flower-pot” method to deprive the animal from REM sleep. In this procedure, the rat rests on a small platform (i.e., the flower pot) surrounded by water and, owing to complete muscle atonia, falls into the water whenever REM sleep starts ( 119 , 387 , 569 ). Awakenings induced in this way are highly stressful and may per se impair later memory performance ( 953 ). However, impairing effects of REM sleep deprivation on memory retention have been also demonstrated with less stressful procedures like gentle head lifting ( 263 ), mild touching ( 596 ), or after pharmacological REM suppression ( 720 , 721 , 1098 , 1108 ). Ponto-geniculo-occipital (PGO) waves which occur associated with REMs in rats and cats have been proposed as a mechanism promoting plastic processes underlying memory formation during REM sleep ( 259 , 260 ) (see sect. IV F ).
The focus on REM sleep as the sleep stage that supports memory has also been criticized by several studies revealing concomitant or even selective increases in non-REM sleep after the animal's exposure to enriched environments or other learning procedures ( 389 , 493 , 495 , 510 , 529 , 540 , 645 , 1092 , 1104 , 1168 ). Non-REM sleep was even proposed as a factor that could explain REM sleep-deprivation-induced memory deficits ( 992 ), and increases in nonREM sleep after fear conditioning correlated with the learned fear response on the next day ( 62 ). In addition, in some cases learning decreased subsequent REM sleep ( 795 , 1029 – 1031 , 1100 ), and this could be accompanied by a concurrent increase in non-REM sleep ( 754 ).
Compared with the findings in rats, evidence for a role of REM sleep in memory processing in humans is surprisingly inconsistent. Most studies failed to find effects of selective REM sleep deprivation on the retention of declarative memories when simple verbal materials (word lists, word pairs, etc.) were used ( 88 , 185 , 192 , 343 , 344 , 729 , 918 , 1094 , 1191 ). Only with more complex declarative materials (meaningless sentences, stories, etc.) did REM sleep deprivation impair declarative memory in some studies ( 350 , 1192 ). In narcoleptics, isolated periods of REM sleep facilitated memory for complex associative information, compared with periods of non-REM sleep or wakefulness ( 1054 ), and learning a topographical map increased subsequent REM sleep in healthy participants ( 365 ). Furthermore, changes in REM sleep patterns were found during intensive study periods (e.g., student exams, see Refs. 1103 and 811 , but see Ref. 559 ). More consistent evidence for an involvement of REM sleep was obtained for tasks with a strong procedural memory component, like learning a foreign language or Morse code (e.g., Refs. 488 , 656 , 657 , 758 , 1249 ). Increases in REM sleep were observed, for example, during training of unfamiliar patterns in motor coordination, like trampolining ( 149 , 150 ) and adaptation to distorted vision by a set of lenses (e.g., Refs. 20 , 232 , 658 , 659 , 1356 , but see also Refs. 19 and 1358 ). REM sleep increased also in infants who learned a head turning response, in contrast to infants who did not learn the response ( 891 ). In a motor finger sequence tapping task, the amount of REM sleep after learning predicted sleep-dependent improvement in this task ( 383 ). Although less clear than in rats, some evidence in humans has also been provided for a REM sleep window of task specific memory processing ( 1103 , 1114 ): memory for the procedural Tower of Hanoi task was revealed to be most strongly correlated with increases in REMs in the second REM episode of postlearning sleep, whereas the improvement in the mirror tracing task correlated with the number of REMs in the fourth REM sleep period. Good learners with higher IQ showed greater increases in REM sleep ( 1114 ). In another study, REM sleep in the last quarter of an 8-h period of sleep, together with the time in non-REM sleep in the first quarter, was highly predictive for learning success in a visual texture discrimination task ( 1157 ).
Compared with declarative learning paradigms, tasks with a strong procedural memory component appeared to be also more sensitive to the detrimental effects of REM sleep deprivation. Karni et al. ( 622 ) were the first to show that sleep after training a visual texture discrimination task substantially reduced discrimination thresholds on the task, indicating a critical importance of sleep for gains in skills that occur offline after training has finished. The overnight reduction in discrimination thresholds was prevented by selective REM sleep deprivation, whereas awakening from non-REM sleep had no effect. Comparing the effects of REM sleep and non-REM sleep deprivation on tasks with strong procedural components (verbal word fragmentation, priming, Tower of Hanoi, Corsi block tapping) and declarative memory tasks (verbal word recognition, visual-spatial learning), Smith and colleagues ( 230 , 1093 , 1094 ) found that total REM sleep deprivation or deprivation of the last two REM episodes of postlearning sleep selectively impaired performance on the procedural memory tasks at the retest session 1 wk later. A similar impairment specific to procedural memory was also revealed after alcohol-induced REM sleep suppression ( 1107 ).
Based on these findings, Smith ( 1094 , 1096 ) suggested that in humans, REM sleep is involved in the processing of procedural memory, whereas REM sleep plays no role in the formation of declarative memories, particularly with respect to simple learning tasks. However, REM sleep deprivation experiments in humans, like in animals, have been criticized due to possible confounding effects of stress on memory formation ( 119 ). In addition, the idea that REM sleep contributes to memory formation has been questioned based on two more fundamental concerns ( 387 , 1076 , 1250 , 1253 ): one was that the large differences in time spent in REM sleep between species, e.g., ferrets spend more than 6 h per day in REM sleep, whereas humans only 2 h, do not translate into any obvious and systematic differences in capabilities to form memories. However, comparisons between species in learning capabilities are per se rather difficult and inconclusive. The second more critical concern is that even the complete absence of any REM sleep, e.g., during treatment with antidepressants, does not lead to any obvious impairment of memory formation (e.g., Refs. 962 and 1252 , but see Refs. 138 and 1310 for discrepant results in rodents), suggesting that at least the overt EEG characteristics of REM sleep are not necessary for successful memory consolidation. On the other hand, the increase in serotonin and catecholamine levels induced by antidepressant intake might compensate for the memory-impairing effects of REM-sleep suppression, as these neurotransmitters have been implicated in memory consolidation processes ( 815 , 842 , 1011 ). To summarize, although REM sleep may benefit procedural memory consolidation, this effect appears to be linked to specific conditions and to underlying, REM-sleep associated biological and molecular mechanisms that are so far unknown.
C. Sleep and the Erasure of Information: Accounts of Emotional Memory
The idea that sleep might be involved in the erasure or filtering of information has been put forward by several authors (e.g., Refs. 210 , 236 , 362 , 369 , 854 ). In particular in 1983, Crick and Mitchison ( 236 ) proposed, based on a neurocomputational model of associative learning, that dreaming during REM sleep helps to forget “parasitic modes” of activity, thus ensuring an efficient mode of operation of the brain during waking. Such parasitic modes of activity particularly occurred after stimulation overload and included “fantasy” (i.e., the net produces far-fetched and bizarre associations), “obsession” (i.e., iterates the same response, irrespective of input) and “hallucination”-like responses (i.e., responds to inappropriate input signals). As a solution to this problem, the authors proposed a “reverse learning” mechanism during REM sleep-dreaming that dampens synaptic weights to reduce the probability of these parasitic activity modes and thereby also enhances the efficacy and storage capacity of the network. Thus, according to this account, dreaming reduces unwanted and bizarre forms of representations in memory, which enhances new learning the next day as well as retrieval of memories acquired before sleep ( 237 ). In simulation studies, repeated unlearning procedures indeed improved the learning capability of the network and retrieval of recently learned patterns, but concurrently weakened more remote memories ( 564 , 1237 ).
Although computational scientists agree in that a mechanism is necessary that limits the strength of synaptic weights in artificial neural networks, empirical evidence for the proposed function of REM sleep, specifically with regard to the removal of “unwanted modes” of activation, is so far lacking. Several authors have reported an influence of REM sleep on emotional reactivity or mood ( 490 , 491 , 1243 ), but a specific influence on “obsessive” or “hallucination”-like behaviors has not yet been tested. In contrast, after awakening from REM sleep, the brain appears to remain in a “hyperassociative” mode, in which weak semantic primes produced distinctly stronger priming effects than during consolidated wakefulness or after awakenings from non-REM sleep ( 1154 ). Similarly, REM sleep awakenings also resulted in a 32% increase in a complex anagram solving task ( 1288 ), and conversely, the need for creative thinking increased subsequent REM sleep ( 730 ). Furthermore, priming before naps filled with REM sleep had a much stronger impact on later creative answers compared with naps without REM or quiet resting, indicating that REM sleep improves creative problem solving instead of reducing creativity ( 163 ). In volunteers asked to voluntarily suppress “unwanted memories,” sleep and particularly REM sleep appeared to counteract this suppression as reflected by an improved retrieval for previously suppressed items, rather than to enhance forgetting of these unwanted memories ( 381 ). Finally, repetitive nightmares, which are highly prevalent in patients suffering from posttraumatic stress disorders (PTSD), do not lead to forgetting of the traumatic event, but are rather associated with increased severity of the disorder and considered a risk factor in the development of PTSD ( 75 , 1240 , 1331 ). Overall, these findings speak against the view posed by Crick and Mitchison ( 236 ).
Of note, in other computational models the mechanisms limiting the strength of synaptic weights in the neural network have been linked to non-REM rather than REM sleep ( 210 ). A recent version of this idea is the “synaptic homeostasis hypothesis” ( 1203 , 1204 ) which will be discussed in section IV B and assumes that a global downscaling in the strength of synaptic connections takes place during SWS to prevent saturation and to reduce place and energy demands, thereby preparing the network for the encoding of new information during succeeding wakefulness ( 565 , 566 ).
If the emotional tone of a memory is considered an “unwanted activation,” then the “sleep to forget sleep to remember” (SFSR) hypothesis recently proposed by Walker and van der Helm ( 1282 , 1284 ) bears some similarities with the ideas about REM sleep by Crick and Mitchison. The SFSR hypothesis assumes that REM sleep after an (aversive) emotional experience strengthens the content of the respective representations in memory, but simultaneously reduces the emotional tone associated with this memory, i.e., reduces the emotional response when the memory is retrieved. The process is not restricted to one night after encoding, but would continue during multiple nights. In depressed patients showing enhanced REM sleep, according to the SFSR hypothesis, this enhancement would bias strengthening of memories towards increased storage of negative contents, while suppression of REM sleep through antidepressants counteracts this bias. The impairment of mood associated with this REM-related process might indicate that the attenuation of emotional tone by REM sleep is not functional in these patients. Similarly, in traumatized patients, increased nightmare frequency would point to a failure to attenuate memory-associated emotions during REM sleep.
Consistent with the theory, several studies have shown that emotional memories are particularly strengthened across sleep ( 560 , 574 , 732 , 861 , 895 , 898 , 1274 , 1276 , 1278 , 1294 ), in particular when containing high amounts of REM sleep ( 179 , 478 , 479 , 1274 ). The enhancing effect of postencoding sleep on emotional memories was detectable even after several years ( 1276 ). Duration and latency of REM sleep significantly correlated with the later recall emotional memories ( 861 ). In the context of fear learning, sleep promoted the generalization of extinction learning ( 878 ), increased intersession habituation to emotional stimuli ( 879 ), and in both humans and rodents, the deprivation of REM sleep impaired fear extinction ( 421 , 1084 , 1133 ). Further support for the SFSR hypothesis was provided by functional magnetic resonance imaging studies indicating that the strengthening of negative emotional memories by sleep is accompanied by a reduced amygdala activation, i.e., a diminished emotional response during retrieval ( 1147 , 1231 ).
However, there are also contradicting findings. For example, two studies have shown that memory recall of negative pictures is less impaired by sleep deprivation after encoding compared with neutral pictures, suggesting a reduced dependency of negative memories on sleep-dependent consolidation processes ( 46 , 1147 ). With respect to emotional reactivity, one study showed that REM sleep-rich sleep amplified subjectively experienced aversion to previously viewed emotional pictures ( 1273 ). Conversely, selective REM sleep deprivation after picture viewing reduced arousal ratings to negative pictures presented again on the next morning ( 704 ). Others found that emotional reactivity decreased across wakefulness, but was preserved during sleep, with the preserving effect on emotional reactivity being specifically linked to REM sleep ( 60 , 481 ). In addition, amygdala responses to correctly recognized emotional objects (with reference to neutral objects) increased rather than decreased after sleep, compared with an assessment after a wake retention interval ( 896 ). The increased amygdala response was accompanied by a stronger connectivity among limbic regions after sleep in this study. Sleep after fear conditioning in humans increases the conditioned response and the associated amygdala activity, with this enhancement being positively associated with postlearning REM sleep (Menz, Rihm, Born, Kalisch, Pape, Marshall, and Büchel, unpublished observation). Sleep likewise facilitated the generalization of implicit fear responses ( 678 ). In animals, REM sleep has been consistently associated with a strengthening of conditioned fear memories (see sect. II B ; for reviews, see, e.g., Refs. 538 , 807 , 1092 , 1094 ). Furthermore, a recent study points towards an involvement of adrenergic activity during SWS instead of REM sleep for the consolidation of emotional information ( 482 ). In conclusion, it is still an open question whether the consolidation of emotional memories actually differs in quality from that of neutral declarative memories, or whether it is the same consolidation process that is simply enhanced or accelerated by the emotional arousal that is attached to the representation at encoding.
D. The Dual Process Hypothesis
The dual processes hypothesis assumes that different sleep stages serve the consolidation of different types of memories ( 428 , 765 , 967 , 1096 ). Specifically, it has been assumed that declarative memory profits from SWS, whereas the consolidation of nondeclarative memory is supported by REM sleep. The hypothesis received support mainly from studies in humans, particularly from those employing the “night-half paradigm.” This paradigm, originally developed by Ekstrand and co-workers ( 341 , 408 , 1340 ), basically compares retention performance across retention intervals that cover either the early or late half of nocturnal sleep. Whereas in the early sleep condition, participants learn (to criterion) the memory tasks in the evening and then sleep for 3–4 h before a later recall test, in the late sleep condition, participants first sleep for ∼3 h (to satisfy the need of SWS) and then are subjected to the learning phase, followed by the late night retention sleep. Due to the circadian rhythm, early nocturnal sleep contains most of SWS, whereas late nocturnal sleep is dominated by REM sleep. Time in stage 1 or 2 sleep usually does not differ between early and late sleep retention conditions. The approach thus allows for comparing the effects of sleep rich in SWS versus REM sleep, elegantly avoiding possible confounding effects resulting from stressful repeated awakenings accompanying standard procedures of selective sleep deprivation. To control possible confounds of the circadian rhythm, the effects of early and late sleep retention periods are typically compared additionally with the effects of wake retention periods that cover the same early and late phases of the night. These two wake control conditions are also necessary to ensure that depth of encoding during the learning phase is comparable between the conditions, because prior sleep is known to influence encoding capabilities ( 328 , 342 , 486 , 515 , 548 , 1190 , 1232 , 1345 ), and learning is preceded by prior sleep only in the late sleep condition, but not in the early sleep condition.
Yarush et al. ( 1340 ) were the first to report a beneficial effect of SWS-rich early sleep on declarative memory (word pairs), compared with retention performance across a REM sleep-rich late sleep or across corresponding wake intervals, and these findings were replicated in a later study of the same group ( 408 ). A similar benefit for declarative (paired-associates) memories was revealed when controlling for circadian influences by placing SWS-rich and REM sleep-rich sleep periods at the same circadian phase, between 3 and 7 a.m. ( 63 ). While these studies quite compellingly showed that declarative memory for neutral materials is enhanced by SWS, emotional declarative memories appear to additionally benefit from REM-rich late sleep ( 1273 , 1274 ). Building on these early studies, Plihal and Born ( 930 , 931 ) not only demonstrated a benefit for declarative memories (word pairs, spatial information) from early SWS-rich sleep, but also demonstrated that late REM sleep-rich retention sleep selectively improved procedural and implicit memories (mirror tracing skill, word-stem priming), compared with corresponding wake-retention intervals. Later studies replicated beneficial effects of late REM sleep-rich sleep on implicit memories (e.g., faces, masked stimuli) ( 1248 , 1277 ), and altogether these findings fit well with the notion of an involvement of REM sleep in procedural and implicit memory processes as revealed by standard REM sleep deprivation procedures (described in sect. II B ).
Improving the effects of early SWS-rich sleep on declarative memory appeared to be overall more consistent with free (or cued) recall measures than with recognition measures of memory ( 265 , 326 , 667 , 966 ; see Ref. 297 , for a review), possibly reflecting that unlike recollection, familiarity based recognition taps to a greater extent implicit components of these memories ( 1343 , 1344 ). Two studies found enhanced recollection (of words) after early, SWS-rich sleep, compared with a late REM-rich sleep or wake intervals, while familiarity-based recognition measures remained unaffected ( 265 , 326 ). In a third study measuring recognition right after free recall, this effect was not replicated, although SWS-rich sleep still enhanced free recall of the declarative materials ( 966 ).
Overall, studies using the night-half paradigm have provided substantial evidence for the dual processes hypothesis, such as hippocampus-dependent declarative memories preferentially profiting from SWS, whereas nondeclarative aspects of memory, such as procedural, implicit, and emotional, additionally profiting from REM sleep ( 121 , 428 ). The hypothesis has been challenged by findings showing that procedural tasks like visuomotor adaptation and visual texture discrimination also benefit from SWS ( 10 , 433 , 581 , 582 ). However, training such skills does not proceed entirely detached from declarative memory mechanisms, especially at an initial stage of training (e.g., Refs. 198 , 533 , 1013 , 1014 , 1042 ). Such declarative components might have mediated the strong benefits for skills from SWS in those studies ( 910 ).
A major weakness of the night-half paradigm is that it ignores possible contributions of stage 2 sleep to memory. Although the amounts of stage 2 sleep were comparable in the early and late sleep conditions of the studies reported above, sleep in this sleep stage may have substantially differed between the two phases, for example, with regard to spindle density ( 440 , 441 , 550 ), heart rate, or levels of neuromodulators like catecholamines and cortisol ( 95 , 124 , 131 , 313 , 554 , 960 ). There is consistent evidence for an involvement of stage 2 sleep and sleep spindles in motor learning ( 139 , 396 , 822 , 862 , 921 ), and memory for a simple motor task is impaired after selective stage 2 sleep deprivation ( 1112 , 1117 ). Training on motor tasks increased the time spent in stage 2 and the density of fast (but not slow) spindles ( 399 , 837 , 920 , 921 , 1173 , 1174 ), and sleep stage 2 duration and fast spindle density also predicted sleep-dependent improvements in a finger tapping task ( 59 , 961 , 1285 , 1286 ). Based on these findings, Smith and co-workers ( 846 , 1114 ) proposed that especially simple motor tasks require stage 2 sleep, whereas complex motor tasks may require REM sleep. However, sleep stage 2 spindle activity and spindle counts were found to similarly correlate with the overnight retention of verbal and visuospatial memories, suggesting that spindles and stage 2 sleep are also involved in declarative memory formation ( 213 , 214 , 1016 ).
E. The Sequential Hypothesis
The sequential hypothesis stresses the importance of the cyclic succession of SWS (or non-REM sleep) and REM sleep for memory formation, with the sleep stages serving complementary functions in this process. The sequential hypothesis originally assumed that in a first processing step during SWS, nonadaptive memories were weakened and adaptive responses were strengthened, whereas during the second processing step during REM sleep, the adaptive memories would be integrated and stored in preexisting knowledge networks ( 23 , 460 , 461 ). A series of studies in rats provided evidence for the hypothesis ( 24 , 25 , 27 , 28 ), for example, reporting high positive correlations between the number of SWS periods followed by REM sleep with memory performance on a two-way active avoidance task ( 698 ). In contrast, the number of SWS periods followed by wakening correlated negatively with performance, providing indirect support for a weakening of memories during SWS, if not followed by REM sleep. In addition, it was argued that SWS led to a global depotentiation of synaptic connections due to the slow EEG frequency as well as the absence of important transcriptional factors. Then, during subsequent REM sleep, the high-frequency EEG and hippocampal theta activity support the strengthening of synaptic connections ( 461 ). Further elaboration of the hypothesis integrated an additional stage of transitional sleep characterized by a sudden mixing of theta and alpha waves with the previous delta waves ( 23 , 929 ). SWS-REM sleep sequences comprising a short period of transitional sleep accurately predicted whether rats learned the active avoidance tasks, whereas in the absence of interposed transitional sleep, rats did not reach the learning criterion ( 26 , 760 , 1254 ).
The sequential hypothesis has also received some support from studies in humans. The overnight improvement on a visual texture discrimination task was best predicted by the time in SWS in the first quarter and the time in REM sleep in the last quarter of the night ( 1157 ). In nap studies, discrimination thresholds in the same task improved only after a longer 90-min nap containing both non-REM and REM sleep, but not after a shorter 60-min nap solely containing non-REM sleep ( 808 ). Similarly, studies adopting the night-half paradigm revealed the greatest improvement in texture discrimination thresholds after a whole night of sleep contained both early SWS-rich and late REM-rich sleep, whereas early SWS-rich sleep per se had only intermediate effects, and REM-rich late sleep was completely ineffective ( 433 ). Experimentally fragmenting sleep such that the cyclic structure was disturbed strongly impaired overnight-retention of words, whereas the same degree of fragmentation did not impair word recall when the sleep cycles were preserved ( 378 ). In the elderly, sleep cycle organization predicted their capacity for overnight-retention of words ( 796 ). It has been also suggested that differences in memory retention between early and late sleep in the night-half paradigm actually reflect differences in the cyclic organization of sleep rather than in the amount of SWS and REM sleep ( 379 ). Indeed, it appears that many findings support the sequential hypothesis, although this hypothesis has rarely been subjected to direct testing.
F. The Active System Consolidation Hypothesis
The hypothesis that sleep supports the formation of long-term memory in an active system consolidation process has been elaborated in several previous reviews ( 293 , 345 , 734 , 828 , 957 , 958 , 984 , 988 , 1284 , 1305 ). The hypothesis integrates aspects of both the dual-process view and the sequential hypothesis. Central to the “active system consolidation” hypothesis is the assumption that memory consolidation during sleep originates from the repeated reactivation of newly encoded memory representations. These reactivations occur during SWS and mediate the redistribution of the temporarily stored representations to long-term storage sites where they become integrated into preexisting long-term memories ( FIGURE 3 A ). The slow oscillations during SWS drive the repeated reactivation of hippocampal memory representations during sharp wave-ripples (SW-Rs; FIGURE 1 B ) in the hippocampus together with thalamo-cortical spindles, which are involved in inducing enduring plastic changes in cortical areas ( FIGURE 3 B ) . Thus reactivation and integration of temporarily stored memories into long-term stores accompany a qualitative reorganization (transformation) of the memory representation (system consolidation) that needs to be stabilized in a synaptic consolidation process assumed to take place during succeeding periods of REM sleep. In claiming that memory consolidation during sleep is an active process, this hypothesis contrasts with accounts that sleep only passively or opportunistically supports consolidation processes mainly by providing a time of reduced interference ( 809 , 1332 ).

A model of active system consolidation during sleep. A : during SWS, memories newly encoded into a temporary store (i.e., the hippocampus in the declarative memory system) are repeatedly reactivated, which drives their gradual redistribution to the long-term store (i.e., the neocortex). B : system consolidation during SWS relies on a dialogue between neocortex and hippocampus under top-down control by the neocortical slow oscillations (red). The depolarizing up phases of the slow oscillations drive the repeated reactivation of hippocampal memory representations together with sharp wave-ripples (green) and thalamo-cortical spindles (blue). This synchronous drive allows for the formation of spindle-ripple events where sharp wave-ripples and associated reactivated memory information becomes nested into succeeding troughs of a spindle (shown at larger scale). In the black-and-white version of the figure, red, green, and blue correspond to dark, middle, and light gray, respectively. [Modified from Born and Wilhelm ( 125 ).]
Compared with the previously discussed hypotheses, the “active system consolidation” account is more concerned with identifying and integrating the neural mechanisms mediating the beneficial effect of sleep on memory consolidation, going far beyond the simple differentiation of sleep stages. In this regard, electrophysiological, neurochemical, and genetic conditions are considered that will be discussed in detail in the next sections. In fact, at a purely behavioral level, numerous studies have demonstrated that sleep after learning benefits declarative ( 17 , 18 , 60 , 63 , 79 , 290 , 326 , 327 , 343 , 348 , 350 , 376 , 408 , 427 , 431 , 478 , 479 , 547 , 560 , 571 , 574 , 592 , 603 , 682 , 732 , 735 , 861 , 898 , 930 , 932 , 959 , 1069 , 1172 , 1192 , 1217 , 1274 , 1276 , 1278 , 1282 , 1302 ) as well as nondeclarative kinds of procedural memory ( 135 , 147 , 164 , 311 , 322 , 374 , 383 , 435 , 447 , 563 , 602 , 631 , 664 , 679 , 733 , 930 , 995 , 1003 , 1286 , 1287 , 1330 ). However, these behavioral findings have but shed little light on the putative processes of memory reactivation and reorganization mediating the consolidation process during sleep.
1. Reorganization of procedural and declarative memories during sleep
With regard to procedural memory, findings that sleep after training of perceptual and motor skills like visual texture discrimination and finger sequence tapping can produce significant improvement (i.e., a gain in skill at a later retesting) have pointed towards an active reprocessing of skill representations occurring during sleep that sharpens the respective representations ( 47 , 57 , 147 , 274 , 275 , 291 , 322 , 383 , 384 , 433 , 582 , 622 , 631 , 664 , 665 , 679 , 762 , 770 , 808 , 810 , 863 , 930 , 1003 , 1153 , 1157 , 1286 , 1287 , 1290 , 1318 ). However, sleep-dependent gains may not equally occur for all types of skills (e.g., Refs. 221 , 1002 , 1015 ). Moreover, recent studies have shown that such gains in skill that are typically measured with reference to the performance level at the end of training, can occur also within a few hours after training in the absence of sleep, and may thus partly reflect a recovery process that is independent of sleep ( 364 , 573 , 1002 – 1004 ). Furthermore, some studies reported that no sleep-dependent gains in procedural motor tasks occur when circadian and homeostatic influences (e.g., time of day, time since sleep) are controlled ( 164 , 991 , 995 ). Also, a mere gain, for example, of speed in finger sequence tapping could be explained solely on the basis of synaptic consolidation processes that strengthen connections formed during training without reorganizing the memory representation. However, convincing behavioral clues for a reorganization of skill representations during sleep come from investigations of sequence-finger tapping skills that show that sleep favors the emergence of an effector independent representation, i.e., sleep benefited pressing the sequence of target keys independent of whether the sequence was tapped with the right or left hand, whereas the sequenced tapping movements per se appeared to benefit also from a wake retention interval ( 218 , 1330 ). Additionally, sleep enhanced sequence-finger tapping performance when learning occurred by observation ( 1234 ) or motor imagery ( 274 ), a further hint towards qualitative changes in the skill representations induced by sleep.
In connection with declarative memory processes, evidence for an active consolidation process during sleep that leads to a qualitative reorganization of memory representations has been provided by studies showing that sleep preferentially supports memory for the “gist” in the learned material, thereby supporting processes of abstraction, inference, and insight. Thus, in adults and children, sleep promoted the integration of newly learned spoken words into existing knowledge networks as measured by a lexical competition task ( 333 , 334 , 530 ; but see Ref. 735 ), with this effect being associated with increased spindle activity ( 1175 ). Sleep likewise promoted grammar-related abstraction processes in language learning tasks in infants ( 469 , 589 ). When participants learned a hierarchy of pairwise presented elements (e.g., A > B and D > E), sleep after learning improved the ability to infer the correct relation between the most distant elements (A > E) ( 345 ). Comparable results were obtained after a nap using relations between different faces paired with the same object, whereby the amount of SWS correlated with the accuracy of relational memory ( 705 , 706 ). Sleep also increased the production of false memories in the Deese-Roediger-McDermott (DRM) paradigm ( 1007 ), in which in the learning phase participants listened to lists of semantically related words (e.g., nurse, patient, hospital, sick, medicine, etc.), whereas the semantic “topic” of the list is not presented (the “critical lure,” e.g., doctor). Compared with wake retention conditions, after sleep subjects showed a greater tendency to “falsely” recall the critical lure, in line with the notion that sleep promotes processes of abstraction and the extraction of the gist information from the list ( 294 , 897 ). However, the effect of sleep in the DRM paradigm was less consistent when recall of the critical lure was tested with a recognition procedure rather than by free recall ( 255 , 296 , 373 ; see Ref. 1159 for an overview).
Using the Number Reduction Task ( 1189 ), Wagner et al. ( 1275 ) showed that sleep facilitates the gain of insight, i.e., explicit knowledge of a hidden structure that was embedded in strings of digits which the subjects had to process before sleep. Subsequent studies of the same group specified that the gain of insight depended on the occurrence of spindle activity during early SWS-rich nocturnal sleep ( 1346 – 1350 ). Very similar results were obtained with an approach combining the classical Serial Reaction Time Task (SRTT) with a so-called “generation task.” On the SRTT, the subject is trained to press as fast and as accurately as possible different keys corresponding to the changing positions of a cue. Unknown to the subject, the changes in the cue position follow a repeating sequence. Typically, during training subjects acquire an implicit knowledge of this sequence as indicated by faster responses to cue positions that follow the sequence compared with responses to random positions. Training does not lead to an immediate formation of explicit sequence knowledge, as the subjects typically cannot deliberately reproduce the SRTT sequence, when explicitly asked to do so right after training (in a generation task). Yet, significant explicit sequence knowledge developed when SRTT training was followed by nocturnal sleep ( 324 , 382 ). Generation task performance in the wake control group in these studies remained at chance level. The sleep-induced extraction of explicit sequence knowledge from an implicitly trained SRTT was particularly pronounced in children, where this extraction process was correlated with enhanced EEG slow oscillation activity during posttraining sleep ( 1321 ). Similarly, in adults performing on a statistical learning task, sleep promoted the abstraction of probabilistic regularities in tone sequences, with this effect being associated with increased SWS during postlearning sleep ( 337 ). Complementary evidence for a neuronal reorganization of memory representations by sleep was provided by studies imaging brain activation during learning and retrieval. In experiments by Takashima et al. ( 1170 ), participants napped for 90 min after studying pictures. Recognition of the pictures was tested 1, 30, and 90 days later. Activation of the hippocampus gradually decreased over time, whereas cortical activation in ventromedial prefrontal areas increased. The duration of SWS during the nap predicted the reduction in hippocampal activity and was also associated with better recognition performance on day 1. A second study of this group confirmed that one night of sleep decreases hippocampal activation and increases activation in neocortex areas ( 1169 ). In another study ( 427 ), the sleep-induced improvement in word pair memories, at a retrieval test 2 days later, was accompanied by an increased functional connectivity between the hippocampus and the medial prefrontal cortex, although contrary to Takashima et al.'s findings, hippocampal activation per se was enhanced at recall in the sleep group. At a follow-up test 6 mo later, the sleep group exhibited increased cortical activation compared with the subjects who stayed awake on the night after word pair learning. Sleep following learning spatial memories of a virtual town produced increased activation in the striatum at a recall test 3 days later, when compared with a group deprived of sleep after learning ( 873 ). Activation in the right striatum positively predicted way finding in the virtual town only in the sleep group, and also functional connectivity between the striatum and hippocampus was modulated by sleep after learning. Similarly, the sleep-enhancing effect of statistical learning ( 337 ) was accompanied by a shift in brain activation from the medial temporal lobe to the striatum ( 336 ). A sleep-induced reorganization of neuronal representations was also revealed for emotional memories (pictures) after 1 night ( 896 ) or 3 days after learning ( 1147 ). In the later study, the reorganization led to increased hippocampal and cortical activation as well as increased connectivity between the medial frontal cortical and hippocampal areas, compared with a control group, which stayed awake on the night after learning. Some of the effects of sleep on brain activation during recall of emotional memories were still observed 6 mo later ( 1148 ). Finally, sleep-dependent changes in brain activity indicative for a reorganization of memory representations have also been reported for procedural tasks like sequences finger tapping and visual texture discrimination ( 384 , 1292 , 1293 ).
2. Selectivity of memory consolidation during sleep
As another key feature, the “active system consolidation” concept implies that memory consolidation during sleep is selective. It is rather unlikely that off-line consolidation strengthens recently acquired memory traces and their synaptic connections globally, because such global and unselective strengthening would inevitably produce a system overflow. In support of this notion, a growing body of experiments indicates that sleep does not equally benefit all memories, although the mechanisms determining whether or not a certain memory gains access to offline consolidation during sleep are currently not well understood.
Several factors have been identified. First, sleep-dependent gains in skills are more robust under explicit learning conditions (i.e., the subjects are aware about the skill to be acquired) ( 383 , 664 , 1285 ) compared with implicit learning conditions ( 385 , 1003 , 1122 , 1128 ). As learning of declarative memories is explicit (and often intentional), these results suggest that explicit encoding favors access to memory consolidation during sleep. Second, the initial memory strength might affect consolidation during sleep, although the available data are not consistent. Stickgold ( 1151 ) proposed that sleep mainly benefits memories encoded at an intermediate memory strength, and that the effect of sleep on memories with varying initial encoding levels follows an U-shaped curve ( 1320 ). Contrasting this view, sleep after learning preferentially strengthened memory for word stimuli weakened by interference learning ( 325 , 343 ) or retrieval-induced forgetting ( 1 ) or for very difficult motor movements ( 679 ). On the other hand, other studies revealed greater benefits from sleep for strongly encoded memories ( 523 , 1172 ) or only in well-performing subjects ( 1038 , 1215 ). Moreover, it is well known that the encoding of emotional events results in stronger memories. Some studies indeed demonstrated that sleep preferentially consolidates emotional over neutral memories ( 574 , 602 , 861 , 896 , 898 , 1276 ); however, others failed to reveal such effects ( 60 , 168 , 732 , 1147 , 1148 ).
A third factor that was consistently found to favor the sleep-dependent strengthening of a memory is the relevance of an encoded memory for an individual's future plans ( 218 , 298 , 380 , 1056 , 1236 , 1319 ). In a sequence-finger tapping task, sleep preferentially improved the goal-related aspects (i.e., the target keys) rather than the movement-related aspects per se (i.e., the tapping executed with specifically the left or right hand) ( 218 , 1330 ). When subjects were promised an extra monetary award after learning (and before sleep) for performing well on one of two equally trained sequences of a sequence-finger tapping task the next day, the sleep-dependent gain on this sequence was greater than for the other sequence that was not associated with reward ( 380 ). Importantly, before the actual retest the next day, the participants were informed that both sequences were equally rewarded to ensure that motivation to perform well was equal for both sequences. Likewise, sleep-associated benefits for declarative memories (e.g., visual-spatial and verbal paired associates) were significantly greater in subjects who were informed before sleep that they would need the materials at a later recall test, than in subjects who were not informed in this way ( 1319 ), and only in the informed subjects later recall performance correlated with SWA during postlearning sleep. A similar selective effect of sleep was reported after learning two sets of picture-location association when only one set was labeled as relevant for later recall testing ( 1236 ). Importantly, in all of these experiments, the expectancy about the later relevance of the memories was induced after the encoding phase, eliminating possible differences in memory strength related to relevance at encoding. Also, when participants were instructed to remember some and to forget other items during learning, sleep preferentially strengthened the to-be-remembered items ( 330 , 381 , 968 ). However, sleep also appeared to counteracted instructed, i.e., “directed” forgetting effects in these studies ( 2 , 330 , 381 ). Similar to the preferential influence on relevant memories, sleep after encoding benefited the memory to perform an intended action at a designated time ( 298 , 1056 ), suggesting an enhancing effect of sleep also on prospective memory for future plans.
Collectively these findings indicate that consolidation processes acting during sleep are driven by motivational factors and specifically strengthen those memories, which are relevant for our goals and future behavior. However, the mechanisms underlying this selection process are unclear. Prefrontal cortex executive functions mediate the processing of anticipatory aspects of behavior and, in collaboration with the hippocampus, these prefrontal regions also regulate the implementation of anticipated memory retrieval as well as the allocation of relevance and expectancies to a memory ( 219 , 511 , 820 , 938 ). In rats, prefrontal cell assemblies that fired during learning when EEG theta coherence between the prefrontal cortex and hippocampus was increased showed a distinctly increased probability to be reactivated during subsequent SWS ( 73 ). Thus theta coherence in the prefrontal-hippocampal circuitry during the encoding of explicit memories might be a critical factor that tags these memories for later consolidation during sleep, with the prefrontal-hippocampal circuitry integrating also emotional and reward-predicting aspects of the encoded events ( 74 , 423 , 782 ). Whether theta coherence likewise mediates tagging that occurred after actual encoding of the memory, i.e., in experiments where subjects were instructed about the future relevance of the learned material after the learning phase ( 380 , 1319 ) remains to be investigated. However, theta-related tagging during encoding might represent a mechanism likewise mediating the preferential consolidation of emotional contents and reward-associated behaviors during sleep, as the network activated by theta also spans brain regions implicated in the processing of emotional stimuli and reward, such as the amygdala and ventral tegmental area, in addition to the prefrontal-hippocampal axis ( 423 , 728 , 887 ).
This view of theta-related tagging of memories integrates a growing number of rodent studies indicating that sleep favors the consolidation of memories that essentially depend on hippocampal functions. In rats and mice, sleep specifically supported consolidation of contextual fear conditioning which is well known to involve the hippocampal function, but did not alter consolidation of cued fear conditioning that does not require the hippocampal function (e.g., Refs. 476 , 165 , but see Ref. 676 ). In a spatial maze task, mice that were sleep deprived after learning shifted from a hippocampus-dependent to a striatum-dependent response strategy (e.g., Refs. 498 and 500 ; for related results, see Refs. 94 , 1106 , 1115 ). Likewise, in studies of novel object recognition, novel place object recognition, and temporal order tasks, retention of the place and time of an event was found to require sleep after the learning phase, whereas the retention of the event per se (item recognition) did not require sleep (e.g., Refs. 92 and 593 , but see Ref. 882 ). Hints that consolidation of memories during sleep depend on hippocampal activation during prior learning have also been provided by human studies using functional magnetic resonance imaging (fMRI) ( 16 , 733 , 968 ). In addition, some human studies revealed that, compared with item memory, context memory, a core function of the hippocampus, is particularly sensitive to the beneficial effects of sleep ( 602 , 732 , 1128 , 1230 ). Interestingly, a recent human study indicated that working memory capacity, a function that in a healthy brain is most closely linked to a cooperative activation of the prefrontal cortex and hippocampus, is strongly correlated with sleep-dependent benefits for hippocampus-dependent declarative memories (word pair associates) ( 374 ). In conclusion, activation of the prefrontal-hippocampal axis in the theta rhythm during the wake encoding process might be the main factor that predisposes a memory for the system consolidation process that takes place during subsequent sleep ( 64 , 782 ).
3. A model of active system consolidation during sleep
The findings discussed above have been used to essentially refine the model of active system consolidation, mainly with respect to memories that are explicitly encoded, via activation of prefrontal-hippocampal circuitry, in the hippocampus-dependent declarative memory system. Basically, the model assumes that during wakefulness the various aspects of an experienced episode are encoded into cortical networks with the different parts of the new memory representation bound together by areas in the medial temporal lobe, especially the hippocampus. During sleep, reactivation of the episodic memory originating from hippocampal networks results in the activation of the different memory parts also at the cortical level, thereby successively strengthening cortico-cortical connections and transforming the temporary representations into long-term memories ( 158 ). Because resources for the strengthening of synaptic connections are less available during SWS, reactivations during SWS might only tag the involved cortical synapses for later strengthening during subsequent REM sleep, in accordance with the sequential hypothesis ( 989 ).
Processes of abstraction, insight, and integration promoted by sleep in this model are thought to be a consequence of reactivation-induced reorganization of memory representation. Thus the repeated reactivation of episodic memory representations during sleep may be capable of identifying and extracting invariant features in the learning material simply because commonalities between acquired memories overall are more frequently reactivated during sleep. Indeed, such extraction processes might facilitate the creation of prototypes and the development of cognitive schemes, i.e., memories less dependent on specific contexts in which they were learned ( 166 , 734 ), and also less sensitive to interference ( 291 , 295 ). Efficacy of this process is probably enhanced as the reactivation of new representations also spreads to closely associated older memory representations, whereby reactivations simultaneously prime the gradual integration of new memories into networks of preexisting old memories. Moreover, reactivation-induced reorganization of memory representations during SWS might enhance the accessibility of memories such that implicitly acquired regularities are strengthened and, after sleep, become accessible to explicit assessment. For this sleep-induced gain of explicit memory, the strengthening of ventromedial prefrontal cortical connections might be particularly important ( 256 , 1321 ).
III. MEMORY REACTIVATIONS DURING SLEEP
The assumption that memories are reactivated during the consolidation phase is an integral part of standard consolidation theory as well as of the “active system consolidation” view of the memory function of sleep ( 418 , 780 , 800 ) (see sect. II F ). Reactivations of memory representations are thought to transform new memories that are still labile and prone to decay into stable memories that are preserved for the long-term. In the standard two-stage memory model, comprised of a fast encoding temporary store (hippocampus) and a slow-learning long-term store (neocortex, see sect. I B3 ), reactivations are regarded as critical for distributing the newly encoded memories to long-term storage sites. These reactivations occur repeatedly and offline (during sleep) to enable the gradual integration of the gist of the new representations into preexisting long-term memory networks, without overwriting these older memories. There is now ample evidence that reactivations of memories occur during sleep.
A. Animal Models
1. reactivation of hippocampal place cells during sleep.
Most reactivation studies in animals examined single unit or multiunit activity from hippocampal place cells in rats. Place cells code for the position of the animal in space relative to certain landmarks, i.e., they fire at substantial rates whenever the animal enters a certain spatial field ( 152 , 868 ). Running along a particular path leads to a specific sequence of place cell firing, which reoccurs when the animal runs along the same path at different times, indicating that the pattern of firing in place cell assemblies is linked to the memory of the spatial environment. Pavlides and Winson ( 893 ) were the first to show that hippocampal place cells exhibit signs of memory reactivation during sleep. They recorded spiking activity from pairs of hippocampal cells with nonoverlapping place fields. When the rats were not allowed to enter the place field of one of the two cells, firing rates of the exposed cell increased relative to the unexposed cell, and this pattern reoccurred during sleep (SWS and REM sleep) after the wake experience.
In a classic study, Wilson and McNaughton ( 1325 ) recorded multiple pairs of hippocampal CA1 place cells, which owing to their overlapping place fields exhibited correlated activity while the rat was running along a track to obtain a food reward. During subsequent SWS, the correlation pattern of these place cells was strikingly similar to the pattern observed during the awake performance. Importantly, the place cell assemblies did not exhibit such firing patterns during sleep before running along the track. Reactivation of firing patterns during sleep after the performance on spatial tasks was likewise obtained in the dentate gyrus ( 1065 ). Based on regression analyses, the activity pattern observed during awake task performance was estimated to explain 10–30% of the variance in spiking activity in the respective place cells during subsequent sleep ( 673 ). Importantly, further studies showed that cells contributing to reactivations are not only coactivated, but a temporal order of spiking in the assembly is also preserved ( 1089 ). In fact, entire spike trains observed during a task performed during prior waking reemerged in the same temporal order during succeeding sleep, with this “replay” running at a faster speed, i.e., in a time-compressed form ( 715 , 716 , 843 ).
Results from initial studies showing reactivation of assembly firing patterns during sleep were criticized because the tested animals were highly overtrained in the spatial tasks ( 538 , 1204 ). Thus, when rats repeatedly perform stereotypical behaviors in a familiar environment, also the firing patterns during pretraining sleep periods become more similar to postsleep periods, and this similarity is reduced when the rat learns a new behavior ( 551 ). This discussion motivated several studies examining reactivations during sleep after rats had engaged in exploratory behavior involving the encoding of new information. These studies uniformly confirmed that signs of assembly reactivation occur in the hippocampus and several other regions of the brain also during sleep after exploration ( 870 , 871 , 985 ). Reactivations could last for more than 24 h, although in the earlier of these studies the data analysis was criticized on methodological grounds ( 1180 ). Neuron assemblies linked to place fields that were longer or more frequently explored during waking showed stronger reactivation during succeeding sleep ( 870 , 871 ). Replay of hippocampal place cell assembly firing during sleep has been observed in many further studies, with the important findings summarized as follows (for detailed reviews, see Refs. 865 , 1163 ): 1 ) during SWS, firing patterns observed during waking are replayed at a much faster (10–20 times) rate, suggesting a time-compressed form of memory reactivation ( 160 , 551 , 606 , 715 , 843 , 1090 ); 2 ) replay activity is typically most prominent within the first 20–40 min of sleep or rest after learning and decays later ( 65 , 673 , 951 , 1065 , 1090 ), although in a few cases signs of hippocampal reactivation persisted for more than 24 h ( 985 ); and 3 ) in hippocampal neuron assemblies, reactivations are mostly observed in conjunction with SW-R ( 673 , 849 , 871 ), which are prominent oscillatory phenomena of the hippocampal EEG and occur in an irregular fashion mainly during SWS but also during quiet wakefulness (see sect. IV D ).
2. Reactivation in nonhippocampal areas
If memory reactivations during sleep promote the redistribution of representations from temporary hippocampal to long-term stores, as it is assumed by the active system consolidation view, neuronal reactivations should also occur in other structures than the hippocampus. In fact, in rats, conjointly with reactivations in hippocampal assemblies, reactivations have been observed in the parietal ( 951 ) and visual cortex during sleep after performance on spatial tasks, whereby replay in the visual cortex tended to slightly follow replay in the hippocampus ( 606 ). Similar to hippocampal replay, reactivation of spatiotemporal patterns during sleep in the medial prefrontal cortex was compressed by a factor of 6–7 ( 361 ). Furthermore, medial prefrontal reactivation correlated with the density of down-to-upstate transition during slow waves in simultaneously recorded cells, K-complexes, and low-voltage spindles in local field potentials ( 608 ), supporting the notion of a functional association between memory reactivations, slow-waves, and sleep spindles supporting consolidation processes during sleep (see sect. IV). In addition, after learning of a new rule in a Y-maze task, reactivation of learning-related patterns in the medial frontal cortex during sleep in distinct bouts mostly followed hippocampal SW-R events with a slight delay of 40 ms ( 926 ), i.e., a temporal relationship consistent with the notion of hippocampal replay guiding neocortical replay. Neuronal replay activity during SWS was also revealed in subcortical regions including the ventral striatum where it was linked to the learning of place-reward associations ( 914 ). Obtaining rewards during learning was associated with strong firing in specific striatal cell firing patterns, which reemerged during succeeding SWS in close association with hippocampal SW-R. Concurrent replay patterns in the hippocampus occurred shortly before striatal reactivations ( 699 , 700 ) and, in contrast to hippocampal replay, reactivation in the striatum did not decay during the 40 min recording period ( 914 ). These findings indicate a leading role of hippocampal memory replay for replay in neocortical and striatal areas. In addition, they are well in line with the idea that redistributions of memory representations during sleep originates from the reactivation of recently acquired memories in the hippocampus, thereby spreading the memory traces to neocortical and striatal sites that may serve as long-term stores.
3. Reactivation during REM sleep
In a few studies, signs of neuronal reactivations have been observed also during REM sleep ( 536 , 544 , 744 , 893 , 935 , 936 ). Interestingly, with the rat growing more familiar with certain parts of a track, respective reactivations of hippocampal firing patterns showed a shift in the phase of the EEG theta cycle such that their occurrence was more likely during troughs of the theta cycle rather than during peaks as typically observed during wakefulness. As spike induced plasticity is known to depend on the theta phase, these data suggest that REM sleep may help erasing episodic memory information once it becomes familiar ( 110 , 935 ). No reactivation was observed when rats were allowed to visit a novel portion of the track. Louie and Wilson ( 744 ) revealed reactivation patterns during REM sleep that showed even greater similarities with patterns that had emerged during REM sleep preceding the performance on the spatial task (circular track), which was possibly related to the fact that the rats were highly familiar with the task which they had performed over several days. Others did not find any hints at experience-dependent neuronal pattern reactivation during REM sleep (e.g., Ref. 132 , 673 ). Indeed, most reactivation studies in rats did not extend their analyses to periods of REM sleep. Thus, although the presence of consistent reactivations of assembly firing patterns during REM sleep is also suggested by modeling approaches ( 521 ), empirical evidence overall remains ambiguous in this regard.
4. Reactivation and behavioral relevance
So far, surprisingly few studies have aimed to examine the functional significance of firing pattern reactivations during SWS for memory consolidation. In this regard, first clues were provided by studies of old rats in which impaired hippocampal reactivation patterns during rest were associated with reduced capabilities for forming spatial memory in the Morris water maze task ( 448 , 449 ). In rats learning goal locations in a spatial task, reactivation of goal-related firing patterns during SW-Rs after learning was predictive for later memory recall ( 335 ). Importantly, blockade of NMDA receptors critical for spatial learning eliminated the tendency to reactivate goal-related firing patterns during SW-Rs, and impaired later memory recall. However, it remained unclear whether reactivations occurred during sleep or waking, as sleep was not recorded in this study.
Direct evidence for a contribution of SW-R-associated reactivations of assembly firing patterns for memory was provided by Nakashiba et al. ( 849 ), who employed a genetic approach to transiently block hippocampal CA3 output, which strongly reduced the occurrence of SW-R events. The blockade resulted in a marked reduction of hippocampal pattern reactivations during sleep and also reduced subsequent retrieval performance in a context fear-conditioning task. The acquisition of spatial memory was likewise impaired when hippocampal SW-Rs in resting rats were suppressed by electrical stimulation of hippocampal afferents ( 340 , 458 ). In the latter study, most (84%) of the single pulse stimulations of the ventral hippocampal commissure occurred in fact during SWS. While these studies provide solid evidence that SW-R events, which act as a carrier wave for neuronal memory reactivations, are behaviorally relevant for memory consolidation during sleep, direct disruption of memory reactivations and its impairing consequences for memory has not yet been demonstrated.
5. Reactivating memories during sleep by cueing
Cueing procedures represent an important tool in examining the functional significance of memory reactivation during sleep. In this approach, contextual cues are associated with the learning materials, which are redelivered during subsequent sleep to reactivate at least parts of the acquired memory representations. Hennevin and co-workers ( 534 , 538 ) were the first to extensively apply this approach to rats. They trained rats on an active avoidance-conditioning task where a mild electrical shock to the ear was associated with a strong aversive foot shock. Redelivery of the mild ear shock during the first six periods of posttraining REM sleep significantly increased recall of avoidance performance after sleep and also increased the time in REM sleep ( 519 ). The delivery of the mild ear-shock was ineffective when the food-shock had been associated with a tone (rather than a mild ear-shock) at training, excluding unspecific effects of stimulus presentation during sleep. Cueing during waking did not affect learning, whereas cueing during SWS and also cueing of old, remote memories during REM sleep acquired 25 days before reduced fear memories ( 517 , 518 ), indicating that cueing of fear memories during sleep strengthen these memories only when cueing is conducted during REM sleep and as long as these memories are fresh. The effects could be connected to cue-specific arousal responses mediated by the mesencephalic reticular formation (MRF) as later studies of this group showed that MRF stimulation during post training REM sleep, but not during waking or SWS, also enhanced memory though in a different task (6-unit spatial discrimination maze) ( 535 ). Recordings of single cells in the hippocampus confirmed that re-exposure to the cue during REM sleep activated firing patterns similar to those observed during training of fear conditioning ( 756 ). Cue-induced firing patterns during SWS were not investigated in these studies. Increased neural responses were also observed after cue reexposure during REM sleep in the medial geniculate body of the auditory thalamus and, for aversive cues, in the lateral amygdala ( 542 , 543 , 755 ). Taken together, these studies by Hennevin's group indicate that learning-induced plasticity can be reexpressed by cueing during REM sleep, whereas expression of conditioned responses was not consistently observed during SWS ( 541 ). Indeed, inasmuch as all of these cueing studies involved a highly emotional, mostly aversive learning component, the REM specificity of the observed effects fits well with the notion that this sleep stage is critically involved in the processing of emotional memories ( 1273 , 1294 ) (see sect. II C ). Most recently, Bender and Wilson ( 75 ) specifically examined whether cueing during non-REM sleep is capable of reactivating hippocampal place cell activity implicated in prior learning. Rats learned by reward to associate an auditory stimulus (sound L or sound R) to either the left or right portion of a linear track (length: 1.5 m). Reexposure to the sounds during subsequent non-REM sleep biased reactivation of hippocampal place cells such that sound L preferentially activated place cells with a left-side place field, and sound R preferentially activated place cells with a right-sided place field. The effect was stronger in the early compared with the later portion of non-REM sleep and did not only pertain to firing rates of individual place cells but the task-related sounds effectively reactivated the temporal structure of the replayed events. Interestingly, cueing did not increase the overall number of reactivations, suggesting a capacity-limiting mechanism for replay activity during sleep ( 626 ).
In sum, the findings in rats have compellingly demonstrated that newly encoded memories are spontaneously reactivated during SWS, whereby reactivations in the hippocampus appear to lead reactivation in other neocortical and striatal regions. Consistent with predictions from computational models ( 613 , 800 ), there is now also evidence that hippocampal activity during SWS (i.e., SW-R, in conjunction with accompanying neuronal reactivations of memory representations) serve the strengthening of these memories, and that these reactivation patterns can be cued by task-related stimuli presented during sleep. Moreover, experimentally enforced memory reactivations during REM sleep were consistently revealed to strengthen emotional memories. However, it remains unclear how exactly this strengthening is achieved by spontaneous or cued reactivations.
B. Human Studies
1. signs of reactivation in pet, fmri, and eeg recordings.
Signs of memory trace reactivation during sleep in humans have been mostly reported using imaging of brain activation with positron emission tomography (PET) or functional magnetic resonance imaging (fMRI). Compared with the multiunit recordings applied in corresponding studies in rodents, these brain imaging techniques suffer from the disadvantage that temporal and spatial resolution is very low. Generally, during non-REM sleep, brain activation and connectivity are distinctly reduced (up to 40%) compared with wakefulness, particularly in prefrontal cortical areas, the anterior cingulum, and several subcortical structures like the basal ganglia ( 134 , 764 , 766 , 768 , 1335 ). However, distinct increases in activity occur associated with sleep spindles or slow waves ( 250 , 1036 , 1132 ). During REM sleep, some brain areas including temporal-occipital cortical regions, the hippocampus and amygdala, exhibit activation comparable to that during waking, whereas activity in others is relatively reduced (e.g., parietal and prefrontal cortices) ( 189 , 253 , 764 , 769 , 1335 ). With regard to memory processes, Maquet and colleagues ( 767 , 912 ) were the first to identify learning-dependent brain activation during sleep using PET. During REM sleep following a 4-h training on a SRTT under implicit conditions (i.e., with the subject being unaware of an underlying regular sequence to be tapped), activation was enhanced in several areas including the bilateral striatum, parietal and premotor cortices, compared with a control group which had performed on a random sequence before sleep, indicating that the activation effects were specifically linked to procedural memory formation. Also, SRTT training changes functional connectivity patterns during subsequent REM sleep ( 707 ). Learning a perceptual skill (texture discrimination) was revealed to reactivate blood oxygen-dependent (BOLD) signal in the trained region of area V1 in the visual cortex during subsequent non-REM sleep, with the magnitude of reactivation predicting improvement visual discrimination skills at retest ( 1351 ).
Clear signs of reactivation during SWS were obtained (by PET) also following hippocampus-dependent spatial learning on a virtual navigation task ( 911 ). Learning to navigate, as expected, activated hippocampal and parahippocampal areas, and the same areas were again activated during subsequent SWS, with the size of hippocampal reactivation predicting navigation performance at a later retest. No signs of reactivation occurred during REM sleep. These findings are convergent with results on replay of firing patterns in hippocampal cell assemblies observed during SWS in rats, and suggest that a similar process of hippocampal memory reactivation occurs also in humans. Learning of face-scene associations induced combined reactivation (in fMRI) in hippocampal and face/scene selective visual cortical areas that occurred particularly during sleep spindles ( 84 ), when functional connectivity between hippocampus and neocortex is generally increased ( 36 ). Notably, reactivations did not only occur in synchrony with spindles, but their size appeared to be also modulated by spindle amplitude, a pattern of findings which is altogether consistent with the view that spindles mediate hippocampo-neocortical interactions during declarative memory processing. Using surface EEG, enhanced EEG coherence, though in different frequency bands, was found during the learning of word pairs and during subsequent SWS, where the coherence effects concentrated on the up-state of the slow oscillations ( 831 ).
Although the available studies provide initial evidence that reactivation of brain areas can be identified in PET, fMRI, and also EEG recordings, more consistently so during SWS than REM sleep, they need to be better characterized using, for example, higher resolution imaging or multivariate pattern classifiers to determine more accurately when and where they occur.
2. Reactivating memories during sleep by cueing
Cueing has also been used in humans to examine the function of memory reactivations during sleep. In early studies using a related approach, participants learned Morse codes and the same codes were acoustically presented again (at a low nonwaking intensity) during subsequent REM sleep ( 331 , 489 ). Reexposure to the codes during REM sleep containing phasic REMs (i.e., acute eye movements) increased performance the next day compared with reexposure during tonic REM sleep without REMs or a no stimulation control condition. In another study, subjects acquired a set of complex rules in the presence of a loud ticking alarm clock and were then reexposed to the ticking sound during phasic REM sleep. At retest after 1 wk, these subjects showed a distinct improvement (by 23%) in memory for the rules compared with control subjects who had not acquired the rules in the presence of the ticking clock ( 1109 ).
Because spontaneous reactivations (of hippocampus-dependent memory) are much more consistently observed during SWS than REM sleep, recent studies have turned towards the examination of cue-induced reactivations during SWS. In a study of ours ( 959 ), we used an olfactory stimulus (the scent of roses) to reactivate visuospatial memories for card-pair locations known to involve the hippocampus ( 1121 ). Odors were used because they do not affect the sleep architecture ( 178 ) and are also known for their strong potency to activate associated memories ( 197 ). The participants learned the card-pair locations in the presence of the odor, with the odor being reexposed during subsequent SWS ( FIGURE 4 A ) , REM sleep or while the subject remained awake. At a later retest, participants recalled significantly more card locations after reexposure of the odor during SWS compared with the other two conditions. The participants were not aware of the odor presentation during sleep. Further controls specified that the memory-enhancing effect of odor exposure during SWS critically depended on whether or not the odor was present during the prior learning phase, indicating that the memory enhancement was caused by a reactivation of odor-associated memories and not simply by unspecific effects of odor-exposure during SWS ( FIGURE 4 B ) . Functional magnetic imaging confirmed that odor presentation during SWS activated the hippocampus, again only when participants had received the odor also during prior learning ( FIGURE 4 E ; Ref. 959 ). Interestingly, hippocampal activation during odor-induced reactivation in SWS was distinctly stronger than during wakefulness, suggesting that during SWS, the hippocampus is more sensitive to stimuli capable of reactivating memories compared with wakefulness. Subsequent experiments (Rihm, Diekelmann, Born, and Rasch, unpublished observation) showed that the improvement in recall was significantly reduced, if the odor presented during SWS differed from that during learning ( FIGURE 4 C ) , and so were also associated increases in EEG delta (1.5–4.5 Hz) and spindle (13–15 Hz) activity during sleep, suggesting that olfactory-induced reactivations of memory representations in the hippocampus can promote oscillatory activity facilitating plastic changes in thalamocortical circuits (see sect. IV, A and C ). Recent experiments show that odor-induced reactivations during sleep can even induce the generation of more creative solutions to a problem encountered before sleep ( 999 ).

Odor-induced reactivations during SWS benefit memory consolidation. A : procedures: participants learned a visuospatial memory task (card-pair locations) in the presence of an odor. During subsequent SWS, they were either reexposed to the same odor serving as a cue to induce memory reactivations, or received an odorless vehicle. After sleep, retrieval was tested (in the absence of the odor). B : odor-induced reactivation of memories during SWS distinctly increased memory for card-pair locations, compared with vehicle condition. In a control experiment, retention of card-pairs remained unchanged when the odor was not administered during learning, excluding unspecific effects of odor exposure on memory processing during sleep. [Modified from Rasch et al. ( 959 ), with permission from American Association for the Advancement of Science.] C : only reexposure during SWS to the same odor as during learning effectively enhanced card-pair memory (congruent odor condition), whereas an odor different from that administered during learning (incongruent condition) was not effective. (Data from Rihm et al., unpublished observation). D : odor-induced reactivations during SWS immediately stabilized memories against interference (induced by learning an interference card-pair task shortly after reactivations during SWS). In contrast and consistent with reconsolidation theory, odor-induced reactivations during wakefulness destabilized memories, as indicated by an impaired card-pair recall when reactivations during waking were followed by learning an interference card-pair task. [Data from Diekelmann et al. ( 295 ).] E : odor-induced reactivations of memories during SWS activated the left hippocampus as revealed by functional magnetic resonance imaging (fMRI). Values are means ± SE: * P ≤ 0.05; ** P ≤ 0.01; *** P ≤ 0.001. [Modified from Rasch and Born ( 957 ), with permission from Elsevier.]
To differentially reactivate individual memory traces during postlearning SWS, Rudoy et al. ( 1018 ), rather than using a global context cue, paired the location of different cards (showing objects and animals) with a characteristic sound (e.g., meow for a cat). During a posttraining nap, only half of the sounds were administered again to reactivate respective place-object associations. At later retrieval, memory for the reactivated associations was significantly better than for the nonreactivated associations. In a subsequent study, reactivation of the characteristic sounds was revealed to be associated with increased activation in the right parahippocampal cortex ( 1235 ). Cueing during sleep was also effective for skill memories. Antony et al. ( 40 ) trained participants to play two different melodies on a piano keyboard. When only one of the melodies was presented again during a period of posttraining SWS, performance on just this reactivated melody was improved at a later retest, compared with performance on the nonreactivated melody. Taken together, in showing that experimentally induced reactivation of declarative and also procedural memories robustly enhance later recall of the memories, these studies demonstrate a causal role of such reactivations for memory consolidation. Thus the cueing of memory during sleep has provided valuable insight into the function of sleep-associated reactivations, stimulating to exploit this approach, in future research, also for specifying the sequels of reactivations for the representational reorganization memories undergone during sleep-associated system consolidation.
3. Memory reactivations and dreaming
It is unclear whether neuronal signs of memory reactivation during sleep are in any way linked to the recall of dreams after awakening from sleep. Highly vivid and emotional dreams are typically reported after awakenings from REM sleep, whereas more thoughtlike dream reports can be obtained after awakenings from non-REM sleep ( 199 , 554 ). Subjectively, reported dreams often cover an extended time period involving a sequence of events, whereas signs of memory reactivation are usually restricted to brief intervals in the range of several 100 ms. However, time perception during dreams might be compressed, as has been reported for replay of hippocampal neuron assemblies during SWS. Nevertheless, although semantic features from past experience are often included, only a very small portion of dream reports (1–2%) incorporate genuinely episodic memories experienced during presleep waking ( 405 , 1050 ). Also, experimental cueing of memories during sleep did not produce specific dream reports of task-related themes ( 959 , 1018 ; Rihm et al., unpublished observation). On the other hand, several experiments showed that specific waking behaviors can influence subsequent dream content ( 1152 ). When participants played an emotionally engaging ski computer game, 30% of dreams reported upon awakenings (from non-REM sleep stage 1 and 2) shortly after sleep onset contained elements related to the computer game ( 1304 ). The same group also reported a link between dream mentation during non-REM sleep and memory consolidation after a nap ( 1303 ), although the effect was based on only four subjects who actually reported task-related mentation.
Overall, there is so far no convincing evidence for a direct link between the reactivation of newly encoded memory representations during sleep as evidenced by the recording of neuronal activity and reported dreams. Although it cannot be excluded that aside from consolidating memory, these reactivations occasionally trigger certain fragments of memory that then are incorporated into dreams.
C. Memory Reactivations in the Wake State
1. animal studies.
Reactivations of hippocampal cell firing patterns occur also during waking when the animal rests after task performance or during brief pauses of active behavior like exploration or running in a maze ( 191 , 268 , 556 , 673 , 870 ; see Ref. 175 for a review). They also occur conjointly with SW-Rs that are observed at somewhat lower rates during wakefulness than during SWS ( 159 , 191 , 870 ). Different from sequenced reactivations in hippocampal assemblies during SWS which always occur in a forward direction, replay of sequences during waking can occur in both forward and backward directions ( 243 , 406 ). In a task requiring the rat to run back and forth on the same elevated linear track, replay in the reverse order occurred mainly at the end of a run, whereas replay in a forward direction transpired in the anticipatory period before a new run ( 288 , 492 ). Wake reactivations were found to be particularly precise when the animal explores a novel environment, and the precision decreases when the animal becomes more and more familiar with the spatial task ( 191 ). In larger experimental environments, both forward and reverse replay can occur over multiple SW-R events thereby covering longer distances ( 268 ). In addition to current learning experience, more remote learning experiences are also reactivated, indicating that wake reactivations do not depend on the actual perceptual input ( 621 ). Also, new path sequences that had never been experienced can be constructed during replay, which could facilitate short-cut learning and the creation of an allocentric cognitive map. However, the exact role of wake reactivations for memory formation and their behavioral relevance has not been thoroughly studied so far. One study reported that both the number of SW-Rs during learning a spatial task as well as during subsequent rest were predictive for later memory performance ( 335 ).
2. Human studies
Signs of spontaneous memory reactivations during the wake state were also observed in humans. FMRI recordings in subjects performing on a vigilance task indicated biased brain activation depending on whether the subjects had performed before on either a procedural serial reaction task or a spatial navigation ( 913 ). Prior performance on the procedural tasks produced relative enhanced activation in striatal and supplementary motor areas, whereas prior navigation performance enhanced activation in temporal lobe regions including the hippocampus. On a shorter time scale, application of multivariate pattern classifiers revealed that MEG responses to sensory inputs (pictures of indoor and outdoor scenes) were replayed during the 5-s delay in a working memory task ( 422 ). The strength of the replay was modulated by the MEG theta rhythm, with the amount of theta phase coordination predicting working memory performance on the pictures. Using an interference A–B A–C paradigm, Kuhl et al. ( 674 ) observed distinct hippocampal activation during learning of the new A–C object associations (interfering with the first learned A–B associations), which was predictive for later remembering the first-learned A–B associations, suggesting that reactivation of old associations during new learning prevented forgetting. Overall, these findings provide first hints that neuronal signs of spontaneously occurring memory reactivations in the wake state can be identified also in humans.
3. Comparing cueing of memories during wakefulness and sleep
Whereas the reactivation of memories during wakefulness, as it occurs for example during rehearsal, can strengthen these memories in the long run ( 623 , 1006 ), the reactivation transfers the representation into an transient unstable state such that memory is in need of reconsolidation ( 470 , 718 , 731 , 844 , 1033 ). Thus, according to the “reconsolidation” concept, memories exist either in an active or inactive state. Consolidation transforms active but unstable memories into passive but stable memories, and reactivation renders these memories again susceptible to interfering influences. There is consistent evidence from animal and human studies that experimentally induced memory reactivations can invoke a transient labilization of respective traces, and also the underlying neural and molecular mechanisms have been partly characterized ( 403 , 404 , 588 , 590 , 639 , 1043 , 1118 ; for reviews, see e.g., Refs. 14 , 844 , 1208 ). Indeed, the destabilization of long-term memories after reactivation might be highly adaptive because it presents the opportunity to update memories with respect to new experiences ( 330 , 513 , 718 , 957 ).
Whether sleep differentially acts on processes of consolidation and reconsolidation is presently not known ( 1034 , 1155 , 1156 , 1285 ). Yet, of more immediate relevance in this context is the question: Does reactivation during SWS like reactivation during waking, transiently destabilize memories? Indeed, in line with the “sequential hypothesis,” it has been proposed that reactivation during non-REM sleep destabilizes memories, which in turn are stabilized during subsequent REM sleep ( 293 , 1156 ). The transient destabilization upon reactivation during SWS could ease the integration of the newly acquired memory into preexisting, neocortical knowledge networks ( 957 ). However, recent experiments appeared to refute these hypotheses. In this study by Diekelmann et al. ( 295 ), memories of card-pair locations were reactivated either during postlearning SWS or wakefulness using a contextual odor cue. To probe stability of the reactivated memory trace, after reactivation (and in the sleep group after being awakened) the participants learned an interference task using the same card pairs but locations that differed from the originally learned task. As expected from reconsolidation studies (e.g., Refs. 844 , 845 , 1043 ), reactivation during wakefulness destabilized memories rendering them susceptible to interference learning, as indicated by impaired memory recall for the originally learned card-pair locations after interference learning ( FIGURE 4 D ) . In sharp contrast, reactivation during SWS had an immediate enhancing effect on the originally learned card-pair locations, although the participants also in this condition learned the interference locations right after reactivation. Note, in this condition memories were reactivated during the first period of SWS not followed by any REM sleep, arguing against the sequential occurrence of REM sleep as another prerequisite for the stabilizing effects of reactivations during SWS. Additional fMRI recordings showed that whereas memory reactivations during SWS mainly resulted in activation of the hippocampus and posterior cortical brain areas, reactivating memories during wakefulness primarily induced activation of the lateral prefrontal cortex ( 295 ).
In showing that the consequences of memory reactivation depend on the brain state with opposing effects induced during waking and SWS, these data refute “opportunistic” theories of sleep-associated memory consolidation ( 809 ) assuming that reactivation-induced consolidation processes do not basically differ between sleep and wakefulness, apart from the fact that sleep protects the processes from external interference (see sect. II A ). Yet, the mechanisms mediating reactivation-induced stabilization and destabilization in the respective brain states are unclear. One factor could be the cholinergic tone which is high during waking but at a minimum during SWS. Cholinergic activity might thus act as a switch that shifts information flow from the prefronto-hippocampal direction prevailing during wakefulness, into the opposite direction during SWS, i.e., from hippocampal to neocortical networks ( 520 , 957 ) (see sect. V B2 ). Related to this, (prefrontal) capacities of explicit encoding and retrieval monitoring available during waking might be critical for whether reactivations actually produce a destabilization of memory traces ( 839 , 1308 ). In combination, these experiments provide emergent evidence for a key role reactivation plays in all phases of memory formation that essentially depends on the brain state.
IV. SLEEP-SPECIFIC ELECTRICAL OSCILLATIONS
Sleep and sleep stages are characterized by specific field potential rhythms of brain activity. Neocortical slow oscillations and SWA, thalamo-cortical spindles and hippocampal SW-R have been associated with processes of memory consolidation during SWS and might support the reactivation and redistribution of memory representations during this sleep stage. Theta rhythms and PGO waves have been proposed to support REM sleep-dependent processes of consolidation and might support enduring synaptic plastic changes during this sleep stage.
A. Slow Oscillations and SWA
1. generation, propagation, and homeostatic regulation.
During human slow wave sleep (SWS), the EEG shows predominant slow wave activity (SWA), which is defined by the 0.5- to 4.0-Hz frequency band and includes the <1-Hz slow oscillations with a peak frequency of 0.8 Hz ( 7 , 830 ). “Delta” activity refers to the 1- to 4-Hz band of SWA. Slow oscillations comprise alterations between periods of neuronal membrane depolarization accompanied by sustained firing (“up-states”) and periods of membrane hyperpolarization associated with neuronal silence (“down-state”). Steriade's group was the first to demonstrate and to provide an in-depth analysis of slow oscillations on the level of intracellular and local field potential recordings in anesthetized cats ( 228 , 1137 , 1138 , 1140 , 1143 – 1145 ). Later studies confirmed that also during natural SWS, cortical neurons are indeed depolarized and fire during the depth-negative (surface-positive) field potential of the slow oscillation half-wave and are hyperpolarized and silent during the depth-positive (surface-negative) half-wave, whereas neurons are depolarized and tonically fire during waking and REM sleep ( 552 , 1143 , 1146 , 1199 ). Virtually every cortical neuron, both excitatory as well as inhibitory neuron, engages in the slow oscillation with the firing patterns showing high synchrony across cellular populations ( 33 , 188 , 1139 , 1146 , 1261 , 1268 ). The widespread synchronization of cortical and thalamo-cortical networks during non-REM sleep is considered the major function of the slow oscillation, providing a global time frame whereby the network is clocked and reset by the hyperpolarizing phase and neuronal processing is limited to the subsequent depolarizing up-phases ( 224 , 282 , 748 , 828 , 832 , 1138 , 1143 ). Indeed, phase-locked electrical and transmagnetic stimulation has revealed the depolarizing up-phase as a period of distinctly enhanced neuronal network excitability ( 83 , 792 , 1200 ). In the scalp EEG, the negative peak of the slow oscillation coincides with the beginning of the down-to-up state transition ( 281 , 993 , 1261 ), whereas the depolarizing phase of sustained firing correlates with the positive EEG deflection ( 33 , 998 , 1268 ). K-complexes during non-REM sleep stage 2 appear to represent isolated slow waves ( 181 ).
The cellular mechanisms of slow oscillation generation are not fully understood. Whereas the up-state reflects some balance between excitatory and inhibitory neuronal activity ( 505 , 1017 , 1074 ), the hyperpolarizing down-state represents a period of disfacilitation that does not contain active inhibition ( 229 , 1146 ). Thus a central question is how up-states are initiated when all neurons are hyperpolarized and silent during the down-state? As a mechanism, hyperpolarization-activated depolarizing currents ( I h ) that depolarize a subset of layer 5 cortical neurons have been discussed ( 1028 ). However, the I h is not strong in cortical neurons and may increase firing only in conditions of increased extracellular K + concentration, e.g., during seizures ( 1194 ). Hence, the more likely explanation is that up-states are triggered by the occasional summation of miniature EPSPs as a residual synaptic activity resulting from stimulus processing during prior wakefulness, and formed mainly by activation of T-type Ca 2+ currents in combination with a persistent Na + sodium inward current ( 1193 , 1198 ). Amplitude and frequency of miniature EPSPs are low under baseline conditions. However, large neuronal constellations, particularly after intense encoding during prior waking, might provide a sufficient number of synapses enabling the generation of repetitive up-states. Moreover, extracellular Ca 2+ concentrations are increased during the down-state, which enhances synaptic efficacy, such that a single spike generated in this condition may effectively excite the whole network ( 238 , 790 ), in particular when such spikes originate from neurons in deep cortical layers with larger numbers of synaptic contacts to other neurons ( 188 ). Once initiated, the up-state is likely amplified by activities of intrinsic currents such as persistent Na + and high-threshold Ca 2+ currents. Contributions of glia cells to the regulation of Ca 2+ concentrations and excitability in neighboring neurons are likely ( 30 , 31 , 506 , 672 ). Synchronization of activity during depolarizing states might be partially achieved via corticocortical glutamatergic synaptic connections, implicating contributions of NMDA and AMPA receptor activation to establish long-range synchrony ( 32 , 360 , 586 ). Induction of the hyperpolarizing slow oscillation down-phase has been mainly linked to synaptic depression, activation of Ca 2+ -dependent and of Na + -dependent K + currents, and the inactivation of persistent Na + currents generally disfacilitating neuronal excitability (e.g., 68, 96, 391, 392, 437, 1053). Recent results suggest that active inhibition is also involved ( 190 , 1027 ), which may be mediated by a particular set of cortical interneurons preferentially firing towards the end of the up-state ( 950 ). During waking and REM sleep, the expression of down-states might be suppressed mainly due to enhanced cholinergic activity in these states.
The slow oscillation is generated in cortical networks and can occur in isolated cortical slices ( 239 , 1028 , 1198 ). Slow oscillations originating from thalamo-cortical neurons ( 586 ) vanish when the thalamus is isolated from cortical inputs ( 1200 ), indicating the slow oscillation is a primary cortical phenomenon ( 993 ). Nevertheless, the slow oscillation of the intact brain likely reflects an interaction between cortical and thalamic networks ( 239 , 1196 ). High-density EEG recordings in humans as well as depth recordings in epileptic patients and cats indicate that the slow oscillation behaves like a travelling wave, which originates most frequently in the frontal regions and propagates towards posterior regions, although other origins and directions of propagation occur ( 188 , 748 , 791 , 841 , 859 , 1261 , 1314 ). Interhemispheric connections contribute to the propagation ( 825 ). EEG-based source modeling located the main origins of the travelling waves in the cingulate gyrus and the left insula ( 841 , 993 ). These estimates roughly correspond with results from PET and fMRI studies likewise indicating frontal as well as midline structures as major sources of slow waves, i.e., the bilateral medial and inferior frontal cortices, precuneus and the posterior cingulate cortex ( 250 , 252 , 557 ). Interestingly, the largest slow waves (>140 μV) were associated with activation in the parahippocampal gyrus, cerebellum, and brain stem, whereas smaller waves were more related to activation changes in frontal areas ( 788 ).
Traditionally, SWA is regarded as a marker of the homeostatically regulated sleep pressure, which increases after prolonged sleep deprivation and decreases from early to late sleep ( 111 , 113 , 994 , 1269 ). It has been argued that the decrease in SWA across sleep reflects differences in the homeostatic regulation between <1 Hz slow oscillations and 1–4 Hz delta oscillations ( 6 , 171 , 1207 ). However, as distinct qualitative differences between both frequency bands have not been confirmed, the decrease in SWA across sleep appears to be most parsimoniously explained by a decrease in the incidence of high-amplitude slow waves ( 1267 ). Independent of the decrease in amplitude, the slope of the slow waves also decreases from early to late sleep, possibly reflecting a decrease in the synchrony and speed of recruitment of neurons at the down-to-up state transition ( 87 , 360 , 994 , 1267 ).
2. The relation between SWA and memory benefits
There is now convergent evidence that SWA and the slow oscillations represent a central mechanism conveying the beneficial effect of SWS on memory consolidation, in particular in the declarative memory system (see sect. II D ). In animals, the encoding and learning of information during waking produced consistent increases in SWA in respective cortical networks during subsequent SWS ( 624 , 1263 ). Thus hemispheric differences in SWA during sleep in rats were related to the preferential use of the left or right paw during the day ( 1265 ). When rats had to rely on their whiskers during the activity period in darkness, subsequent SWA was higher in the somato-sensory cortex compared with a situation in which additional light was available during the active period ( 1341 ). In birds, sensory deprivation of one eye while watching a documentary about birds increased SWA and the slope of slow waves only in the hyperpallium (a primary visual region) connected to the stimulated eye ( 726 ).
In humans, intense learning of declarative memories (word pairs) enhanced amplitudes of the slow oscillation up-states as well as coherence in the SWA frequency band during succeeding SWS ( 829 , 831 ). Also, slopes of the down-to-up state of the slow oscillations were steeper after learning. Regarding procedural skills, training on a visuomotor adaptation task increased the amplitude of slow waves during subsequent SWS ( 581 , 582 ). The increase was locally restricted to the motor areas mainly involved in prior training and correlated with the overnight improvement on the task. Increases in local SWA were even observed when learning took place in the morning, suggesting that the training-induced changes in SWA do not depend on the time between training and subsequent sleep ( 751 ). Conversely, arm immobilization during daytime resulted in reduced SWA over the contralateral motor cortex ( 581 ). Correspondingly, reducing SWA during sleep by the presentation of tones was revealed to suppress the sleep-dependent improvement in visuomotor adaptation skills ( 691 , 692 ) and also in texture discrimination skills ( 10 ).
Several studies examined the effects of repetitive transcranial magnetic stimulation (rTMS) as a tool to directly induce synaptic potentiation, on subsequent SWA. Application of 5 Hz rTMS over the motor cortex induced an immediate potentiation of the TMS-induced cortical response which was followed by a marked (40%) increase in SWA in the same cortical region during subsequent sleep ( 580 ). Paired associative TMS stimulation (PAS) before sleep produced increases or decreases in subsequent SWS, depending on whether the protocol successfully induced long-term potentiation-like increases or long-term depression-like decreases in cortical excitability as measured by motor evoked potentials ( 583 ). In another study, similar effects of PAS stimulation on SWA were associated with local changes in slow spindle activity ( 85 ). Changes in frontal SWA after PAS appeared to extend even into succeeding REM sleep ( 443 ). Overall, these findings support the view that SWA (as well as the amplitude and down-to-up state slope of the slow oscillation during SWS) reflect the intensity and amount of encoding during prior wakefulness, with some studies also indicating an association of these measures with later retrieval.
Direct evidence for a causal role of slow oscillations on sleep-dependent memory consolidation is provided by studies experimentally inducing slow oscillations by transcranial direct current stimulation (tDCS; FIGURE 5 A ). In humans, tDCS that oscillated at a very low frequency (0.003 Hz; 30-s on/30-s off) and was applied to the prefrontal cortex during early nocturnal non-REM sleep, increased endogenous SWA, and produced a significant improvement in the overnight retention of word pair memories ( 785 ). Effects on word pair memories were even more consistent with tDCS oscillating at 0.75 Hz, i.e., a frequency mimicking the endogenous slow oscillations ( FIGURE 5 B , Ref. 783 ). This type of stimulation applied during early non-REM sleep specifically enhanced <1 Hz slow oscillations and additionally increased frontal slow spindle activity (10–12 Hz). tDCS at the same frequency (0.75 Hz) during late REM-rich sleep was ineffective in enhancing both endogenous slow oscillations and overnight retention of word pairs. Further controls ensured that the effects depended on the frequency of the oscillating stimulation and on the brain state ( FIGURE 5 C ) : tDCS at the 5 Hz theta frequency during early non-REM sleep had an immediate suppressing rather than enhancing effect on endogenous slow oscillation and frontal slow spindle activity, and impaired overnight retention of word pairs ( 784 ). Applying the 0.75 Hz slow oscillatory stimulation during wakefulness induced a widespread increase in theta (4–8 Hz), rather than slow oscillation activity, and this increase in theta activity was associated with a significant improvement in the encoding of declarative memories, rather than affecting retention of these memories ( FIGURE 5 D , Ref. 643 ). These results agree with findings that reveal increased cortical excitability following oscillatory electrical stimulation in the wake state ( 83 , 484 ) and provided initial hints at a causal role of EEG theta activity for the encoding of new memories in humans ( 646 , 647 , 687 ). In fact, combination changes observed after tDCS at different frequencies seem to indicate that the cortical networks oscillating at the theta frequency during encoding of hippocampus-dependent memories are functionally linked to the networks that oscillate at the slow oscillation frequency during subsequent SWS to consolidate these memories.

Probing the functional relevance of slow oscillatory activity for memory processes by transcranial direct current stimulation (tDCS). A : procedures: participants learned declarative and nondeclarative tasks before sleep and recall was tested in the next morning. During early postlearning non-REM sleep, tDCS oscillating at different frequencies was applied via electrodes attached bilaterally over the prefrontal cortex and to the mastoids. In a sham control condition, no current was applied. B and C : effects of tDCS depend on frequency of the oscillating stimulation. B : tDCS during non-REM sleep oscillating at the 0.75 Hz slow oscillation frequency (SO-tDCS) increased endogenous slow oscillation activity (0.5–1 Hz) at all recording sites and slow frontal spindle activity (8–12 Hz), and these effects were associated with an enhanced retention (consolidation) of declarative memory (for word pairs) across sleep, compared with the sham condition. [Data from Marshall et al. ( 783 ).] C : in contrast, tDCS oscillating at 5 Hz (theta-tDCS) decreased slow oscillation activity at all recording sites and slow frontal spindle activity, and these effects were associated with an impaired retention of declarative memory across sleep. [Data from Marshall et al. ( 784 ).] D : effects of SO-tDCS depend on brain state: when applied during waking (rather than during non-REM sleep), tDCS induced a widespread increase in 4–8 Hz theta and 16–14 Hz beta activity, rather than slow oscillation activity, and these increases were associated with an enhanced encoding of declarative memory (for words), particularly in later learning trials (R5, R6), whereas consolidation across the wake retention interval remained unaffected (not shown). Values are means ± SE: * P ≤ 0.05; ** P ≤ 0.01. [Data from Kirov et al. ( 643 ).]
B. The Synaptic Homeostasis Hypothesis
1. the concept.
An influential concept proposed by Tononi and Cirelli ( 1203 , 1204 ) assumes that the slow waves of SWS serve primarily to globally down-scale the strength of synapses that were potentiated in the course of encoding of information during prior waking. According to this concept, linear downscaling across synapses enhances memory indirectly as this process nullifies the strength of connections that were only weakly potentiated during wakefulness leading to the improvement of the signal-to-noise ratio for more strongly encoded memory representations. Synaptic downscaling can be considered a nonspecific process complementing active system consolidation during sleep ( 293 ).
The synaptic homeostasis hypothesis originated from Borbely's “two process” model of sleep ( 5 , 8 , 111 , 112 ), assumes that, apart from a circadian process C, sleep and specifically SWS is regulated by a homeostatic process S, which rises during waking and declines during sleep. The most important marker of process S is SWA during non-REM sleep, which reliably increases as a function of time awake and shows an exponential decrease in the course of subsequent non-REM sleep. The synaptic homeostasis hypothesis basically links process S to processes of synaptic plasticity ( 1203 , 1204 ). It relies on four key assumptions. First, it is assumed that wakefulness in general is a state of information intake and encoding that induces processes of LTP in cortical networks resulting in a net increase in synaptic weights. Second, it is assumed that SWA is a direct marker of the amount of synaptic potentiation during prior wakefulness such that “the higher the amount of synaptic potentiation in cortical circuits during wakefulness [is], the higher [is] the increase in slow wave activity during subsequent sleep” (p. 144 in Ref. 1203 ). Third, it is assumed that SWA is implicated in the downscaling of synaptic weights. As synapses are predominantly potentiated during wakefulness, such process would inevitably lead to increasing energy and space demands for permanently increased synaptic weights, ultimately saturating the network thus impairing further encoding of information. SWA and in particular the <1 Hz slow oscillations are assumed to proportionally downscale the potentiated synapses by a long-term depression-like mechanism, as neuronal firing at frequencies <1 Hz is known to preferentially induce long-term depression ( 630 , 789 ). Moreover, down-states of neuronal silence following up-states of enhanced firing increase the probability that presynaptic input is not followed by any postsynaptic output, i.e., a mechanism leading to further depotentiation of the network. Also, the neuromodulatory milieu during SWS is characterized by low levels of acetylcholine, norepinephrine, and serotonin and therefore favors processes of depotentiation. The downscaling process, and in parallel the propensity for SWA, is self-limiting as synapses are gradually depotentiated, reaching a constant homeostatic level at the end of sleep. A computational model confirmed that a decrease in synaptic strength can fully account for the gradual decrease of SWA from the beginning to the end of the sleep period ( 360 ). Specifically, the model predicted a decrease in the incidence of high-amplitude slow waves, a decrease in slope, as well as an increase in the number of multipeak waves from early to late sleep, which was confirmed in related animal and human studies ( 994 , 1269 ). Fourth, the synaptic homeostasis hypothesis assumes that memories are enhanced by sleep as a by-product of synaptic downscaling. During learning, correct (signal) as well as erroneous information (noise) is encoded, although the latter at a weaker strength. The proportional downscaling of synapses during SWS reduces the weights of weaker synapses below a threshold, making them completely ineffective, whereby the signal-to-noise ratio and the subsequent recall of the memory representation is enhanced.
The concept, and specifically the association of synaptic potentiation during wakefulness and subsequent SWA, was confirmed by several studies measuring markers of synaptic plasticity like plasticity-related genes [brain-derived neotrophic factor (BDNF), activity-regulated cytoskeleton-associated protein (arc), Homer, nerve-growth factor-induced gene A (NGFI-A)] ( 584 ). In rats, learning a reaching task increased the protein expression of two activity-dependent genes c- fos and arc in the motor cortex and increased subsequent SWA in the same brain region ( 510 ). Suppressing expression of LTP-related genes in rats by chronic lesion of the noradrenergic system produced a strong reduction in the homeostatic SWA response (see, e.g., Refs. 205 and 457 , for similar results in flies). Levels of postsynaptic glutamatergic AMPA receptors containing the glutamate receptor (GluR1)-1 subunit reliably indicate synaptic plasticity and were revealed to be high during wakefulness and distinctly lower (by 40%) during sleep ( 1264 ). Correspondingly, results from a recent study suggest that discharge patterns of pyramidal neurons during SWS promote the removal of synaptic Ca 2+ -permeable AMPA receptors in the somatosensory cortex of juvenile rats ( 701 ). Procedures assumed to induce local net increases in synaptic potentiation (e.g., the cortical application of BDNF and KCl) induced cortical spreading depression ( 81 ) and increased SWA during subsequent sleep, whereas BDNF receptor inhibition decreased SWA ( 366 , 367 ). Importantly, in demonstrating that the encoding of information during waking enhances subsequent SWA in specific brain regions, these studies provide compelling evidence that SWA is regulated locally in addition to its global regulation by brain stem and diencephalic structures ( 672 , 859 , 975 , 1267 ).
Supplementary evidence for sleep-associated synaptic downscaling was obtained in two recent studies measuring synaptic growth. Using staining techniques in fruit flies, Bushey et al. ( 156 ) showed that synapse size or number increased after a few hours of wakefulness and decreased only when flies were allowed to sleep. Increased synaptic growth due to an enriched wake experience (12 h spent together with 100 other flies) led to an increase in sleep with the time spent asleep after enriched experience being negatively correlated with spine density, suggesting that sleep renormalized synapses after potentiation during wakefulness. In juvenile mice in vivo, two-photon microscopy in the sensorimotor cortex revealed a net decrease in spine growth across periods of sleep, although there was also significant spine growth at the same time ( 772 ). In adult mice, no similar changes were observed.
Further support for a net increase in synaptic weights across the wake period and a decrease across sleep derives from measures of cortical excitability and actual firing rates which should be increased with net increases in synaptic strength. Indeed, in rats, slope and amplitude of the electrically evoked cortical potential as indicators of synaptic strength progressively increased during sustained wakefulness and decreased after sleep, and these changes were correlated with SWA ( 1264 ). In humans, cortical excitability as measured by a combined TMS/EEG study was increased after sustained wakefulness and decreased after sleep ( 71 ), although another study reported a decrease in TMS-induced cortical excitability after 40 h of sleep deprivation ( 445 ). Firing rates of cortical neurons were high after periods of sustained wakefulness and decreased during sleep, with the decrease correlating with SWA during sleep ( 1268 ). Miniature excitatory postsynaptic currents (mEPSC) are considered residual activity resulting from prior potentiating of synapses in the course of information encoding. Frequency and amplitude of mEPSCs were increased in the frontal cortex slices of mice and rats after prolonged wakefulness compared with slices obtained after sleep ( 740 ).
There is also evidence that, together with increased net synaptic potentiation, wakefulness leads to a gradual increase in energy demands, e.g., glucose uptake, of the brain. Indeed, cerebral metabolism measured by 2-deoxyglucose uptake increased after periods of wakefulness and decreased after sleep in mice ( 1265 ). However, decreases in cerebral metabolic rates after prolonged waking have also been observed in rats ( 363 ). In humans, cerebral glucose uptake measured by PET did not appear to be decreased when measured 2–4 h after sleeping ( 157 ). Moreover, decreases rather than increases in metabolic rates were observed after 24 h of sleep deprivation ( 1186 , 1336 ), although it cannot be excluded that distinctly extended periods of wakefulness trigger separate compensatory processes characterized by the occurrence of local slow waves in the waking state and by reduced metabolic demands ( 1204 , 1267 ).
2. Critical issues
Although the synaptic homeostasis hypothesis integrates a wide variety of findings, especially on SWA, concerns have been raised about both the concept of downscaling in general, and specifically about how the theory explains sleep-dependent memory benefits. In light of clear evidence that learning during wakefulness relies both on LTP and LTD-like mechanisms (e.g., Refs. 222 , 629 ) “ … it is thus most improbable that sleep need - to the extent this is determined by learning - is determined solely by Hebbian LTP (or any other single form of synaptic plasticity)” (p. 4 in Ref. 410 ). Also, molecular markers of synaptic potentiation like arc, BDNF or Calmodulin-dependent-kinase (CaMK) IV are involved in both the potentiation and depression of synaptic strength or other forms of non-Hebbian scaling ( 1026 , 1067 ), questioning that the relative increase and decrease of these markers across sleep and wakefulness, respectively, is actually related to one specific form of synaptic plasticity. Furthermore, there is evidence that protein synthesis involved in LTP stabilization is enhanced during sleep, with the rate of synthesis linked particularly to SWS ( 848 , 956 , 1245 ) (see also effects of sleep on ocular dominance plasticity discussed in section VII A3 ). Recently, Chauvette and colleagues ( 187 ) showed in vivo that somatorsensory evoked potentials in cats were enhanced after a short period of SWS (about 5–10 min) compared with the previous wake period. Further experiments in vitro confirmed that the SWS-related enhancement in synaptic strength is a calcium-dependent postsynaptic mechanism which requires (i) the hyperpolarizing down-state of the slow oscillation and (ii) the coactivation of AMPA and NMDA receptors, suggesting that SWS is linked to synaptic potentiation rather than down-scaling ( 118 ). Related to that, although stimulation at the ∼1 Hz slow oscillation frequency typically induces LTD in vitro, in vivo such stimulation can remain ineffective or even induce LTP (e.g., Refs. 497 , 919 ). In fact, the original concept of synaptic scaling as a self-tuning process of neuron networks assumes that periods of reduced synaptic activity, like sleep, produce net up-scaling rather than downscaling of synapses ( 409 , 1219 , 1220 ).
The memory-benefit from sleep, according to the synaptic homeostasis theory, reflects an increased signal-to-noise ratio for the memorized representation that occurs as a byproduct of proportional synaptic downscaling, shifting weakly potentiated synapses below a threshold thus nullifying their weight. So far, there is no experimental data supporting the existence of such a threshold, nor for the assumption that synaptic scaling in the cortical network is proportional ( 293 ). Compared with older memories, new memories appear to be generally more labile, i.e., linked to weak synaptic weights and thus would be at a greater risk of being erased during sleep. This prediction contradicts experimental findings pointing towards the preferential consolidation of weaker over stronger representations during sleep ( 325 , 343 , 344 , 375 , 679 ). In classic interference paradigms, learning a second list of words after a first list weakens memory for the first list learned due to retroactive interference. Contrary to predictions from the synaptic homeostasis theory, the benefit from sleep for the first (weaker) list of words learned was significantly stronger than for the second list learned ( 325 , 343 ). There is indeed a lack of any behavioral data indicating sleep-associated forgetting of irrelevant memories ( 293 ).
Signs of reactivations of neuronal memory representations observed during SWS, in the synaptic homeostasis view, reflect ongoing activity in hypermetabolic traces induced by intense learning in these networks, without any relation to memory consolidation ( 869 ). This contrasts with findings indicating that an external triggering of memory reactivations during sleep enhances memory performance the next day ( 295 , 959 , 1018 ) and that spontaneous memory reactivations during sleep correlate with later memory performance ( 335 , 448 , 449 ). Consequently, findings of a sleep-dependent reorganization of neuronal memory representations also represent a challenge to the synaptic homeostasis theory inasmuch as this implies that some parts of a representation undergo up-scaling during sleep while others are down-scaled (see sect. III). As any synaptic downscaling per se cannot explain the memory consolidating effects of sleep, reactivation-based consolidation has been proposed as a core mechanism driving active system consolidation during SWS, including basically local processes of up-scaling and synaptic strengthening. However, these processes might be complemented and even enhanced by an unspecific process of synaptic downscaling that acts on a global scale to maintain overall synaptic homeostasis ( 293 ).
3. SWA enhances subsequent encoding
Importantly in the synaptic homeostasis theory, sleep-associated synaptic down-scaling primarily serves to renormalize synaptic weights in networks that, in the course of the encoding of information during prior wakefulness, were potentiated to close to saturation. Therefore, rather than support the consolidation of memory, synaptic down-scaling during SWS is expected to ease the encoding of new information during subsequent wakefulness. Indeed, there is convergent evidence for such action in humans and rats, i.e., an impairment of learning when they were deprived of sleep ( 195 , 328 , 500 , 634 , 759 , 1291 , 1345 ). Fittingly, in rats, sleep deprivation also hampered the induction of LTP in the hippocampal regions ( 170 , 778 , 1161 , 1179 ), the expression of plasticity-related genes ( 496 ) as well as excitability of the hippocampal neurons ( 801 , 802 ). Selective deprivation of REM sleep appears to be sufficient for producing an impairment in LTP induction ( 269 , 270 , 596 , 636 , 743 , 970 , 1010 ). In humans, the capacity to encode episodic memories deteriorates across the day and is restored after a period of sleep ( 759 ). Prior sleep deprivation impairs verbal learning ability and reduces temporal lobe activation during learning ( 328 , 329 ). Interestingly, the ability to encode pictures after a nap was already substantially diminished when SWA during napping was selectively attenuated by contingent mild acoustic stimulation leaving the gross sleep architecture intact ( 1232 , 1233 ). The impairing effect on picture encoding was accompanied by reduced hippocampus activation during learning. Surprisingly, no change in activation in relevant neocortical areas was found, and suppressing SWA also did not impair the learning of a motor skill task. Complementary effects, i.e., enhanced encoding on hippocampus-dependent declarative tasks in the absence of changes in motor skill learning, were revealed after enhancing sleep SWA by transcranial direct current stimulation (oscillating at the 0.75 Hz slow oscillation frequency) ( 39 ). However, no signs of improved encoding were revealed after a drug-induced enhancement of SWA by sodium oxybate ( 1297 ). After 36 h of total sleep deprivation, encoding of emotional pictures was strongly impaired, with this effect sparing negative emotional pictures ( 1291 ). Interestingly, amygdala responses during the viewing of aversive pictures increased after 35 h of sleep deprivation ( 1345 ). Collectively, these initial data corroborate the idea that SWA during sleep refreshes capacities for the encoding of information possibly by a synaptic down-scaling-like mechanism. It remains unclear why the effect apparently predominates in hippocampal networks that encode information in declarative tasks, although the hippocampus itself does not generate slow oscillations ( 597 ).
C. Spindles
1. spindle generation, fast and slow spindles.
Spindle activity refers to regular EEG oscillatory activity which occurs in a frequency range between ∼10 and 15 Hz and expresses in human non-REM sleep stage 2 as discrete, waxing and waning spindles lasting 0.5–3 s ( 440 ). Spindles occurring during stage 2 sleep low-voltage EEG can be temporally locked to a vertex sharp wave or a K-complex. Spindles are also present during SWS and superimposed on delta activity, and then form less clearly discrete spindles ( 38 , 53 , 303 , 426 , 440 , 442 , 785 , 1083 , 1221 , 1355 ). Although in the beginning of SWS spindle activity can reach levels similar to those in stage 2 sleep, on average spindle activity in SWS is lower than during stage 2 sleep. There is a reciprocal relationship between spindles and SWA such that while SWA progressively decreases across nocturnal sleep in humans, sleep spindles and power in the 12–15 Hz (“sigma”) band tend to increase ( 11 , 442 ). Sleep deprivation typically reduces spindle activity during subsequent recovery sleep, together with an increase in SWA ( 114 , 300 – 302 ), and similar reciprocal changes, i.e., decreases in SWA conjoint with increases in spindle activity, are observed after administration of GABA A receptor agonistic drugs (see sect. V A2 ).
Spindle activity originates in the thalamus from mutual interactions between GABAergic neurons of the nucleus reticularis ( 670 ) which function as pacemaker, and glutamatergic thalamo-cortical projections which mediate the synchronized and widespread propagation of spindles to cortical regions ( 227 , 440 , 1139 , 1195 ). The isolated reticular thalamic nucleus is indeed able to generate spindles, whereas the thalamus isolated from the reticular thalamus is not ( 1141 , 1142 ). Thalamic generation of spindles is linked to activation of T-type calcium channels ( 45 , 67 , 1162 ) and calcium-dependent small-conductance channels ( 1326 ). Within neocortical networks, spindle activity is probably associated with a massive calcium influx into pyramidal cells ( 1061 ). Repeated spindle-associated spike discharges efficiently triggered LTP in neocortical synapses in in vitro models ( 1012 , 1197 ). In vivo, synchronous spindle activity occurred more reliably in neocortical synaptic networks that had been previously potentiated through tetanic stimulation ( 1315 ). Correspondingly in humans, the local expression of spindle activity during non-REM sleep increased or decreased depending on whether LTP-like or LTD-like plasticity had been induced through transcranial magnetic stimulation (TMS) before sleep ( 85 ). These observations suggest that spindles occur preferentially in potentiated synaptic networks and may contribute to maintaining this potentiation.
Studies in humans have consistently revealed the presence of two kinds of spindles: fast spindles (∼13–15 Hz) show a more widespread distribution concentrating over the central and parietal cortex, whereas slow spindles (∼10–12 Hz) show a more focused topography over the frontal cortex and are more pronounced during SWS than stage 2 sleep ( 34 , 440 , 826 , 1184 ). The two types of spindles differ in many aspects, including their circadian and homeostatic regulation, pharmacological reactivity, as well as their age-related changes ( 440 ). With the use of EEG and MEG, neocortical sources of the classic fast spindles have been located in the precuneus, and for slow spindles in the prefrontal cortex (Brodman areas 9 and 10) ( 34 , 763 ). Combined EEG/fMRI recordings revealed that both spindles are associated with increased activity in the thalamus, anterior cingulate, and insula cortices ( 1036 ). However, slow spindles were associated with increased activation in the superior frontal gyrus, whereas fast spindles recruited medial frontal, midcingulate, sensorimotor, and supplementary motor cortical areas. Importantly, fast spindles were also associated with increased activation in the hippocampus, suggesting a particular relationship between classic fast spindles and hippocampus-dependent memory processes during sleep. It has been suspected that slow spindle activity reflects predominant coupling among cortical networks, whereas fast spindles may be more closely related to thalamocortical coupling ( 317 ). Indeed, optogenetic stimulation of reticular thalamic neurons can induce spindles in the neocortex, that are not accompanied by thalamic spindles ( 507 ), suggesting an active role of the neocortex in the expression of spindle oscillations. Despite such evidence, it remains a matter of debate whether fast and slow spindles actually reflect different neural processes or are simply the modulation of a single spindle generator ( 440 , 788 ). The picture is complicated by recent MEG data as well as intracranial recordings from neurological patients that identified multiple local generators for neocortical spindles ( 38 , 52 , 276 , 859 ). Importantly, this research revealed that in the neocortex, single spindles typically express as a local phenomena and are restricted to specific regions and circuits, regardless of whether or not they are synchronized in phase with central thalamic spindle generation.
2. Relationship between spindles and memory
Many studies in humans and animals have indicated a robust association between spindle activity and memory processing during sleep. Effects appear to be particularly consistent for the classic fast spindles. However, not many studies differentiated both types of sleep spindles. Intense learning of declarative memories (word pairs or virtual maze) increases the number of spindles (12–15 Hz) during subsequent sleep, particularly in the early part of the night ( 432 , 812 ). In another similar study, only participants who exhibited enhanced spindle activity (11.5–16 Hz) after word pair learning (in comparison with sleep after a non-learning control session) also showed a significant overnight improvement of verbal memory ( 1037 ). Encoding of a difficult list of abstract words produced an increase in the power and density of spindles (11.27–13.75 Hz) compared with sleep after encoding a list of easier, concrete words ( 1044 ). In rats, robust increases in sleep spindles (12–15 Hz) were observed after learning odor-reward associations ( 354 ) and after avoidance training ( 397 ). Furthermore, indicators of sleep spindle expression consistently correlated with the amount of overnight retention of declarative memories ( 86 , 213 , 214 , 235 , 446 , 562 , 822 , 1016 , 1024 , 1037 , 1044 , 1057 ). Interestingly, spindle activity (11–15 Hz) also correlated with signs of overnight lexical integration of newly learned information, suggesting that spindles contribute to the integration of new memories into existing neocortical knowledge networks ( 1175 ). Moreover, spindles (13–15 Hz) during a postlearning nap predicted sleep-dependent memory improvement for contextual aspects of episodic memories known to most closely depend on the hippocampal function ( 1230 ). Using combined EEG/fMRI recordings, Bergmann et al. ( 84 ) observed conjoint reactivations in relevant neocortical and hippocampal regions that occurred in temporal synchrony with spindle events (12–14 Hz) during non-REM sleep after learning of face-scene associations. The strength in reactivations covaried with spindle amplitude. Together these findings provide first hints that spindles are implicated in the hippocampo-neocortical exchange of memory information mediating active system consolidation during sleep. Interestingly, spindle activity following exposure to a novel spatio-tactile experience in rats predicted immediate early gene activity (Arc expression) in the somatosensory cortex during later REM sleep, suggesting that neocortical parts of representations become tagged for later synaptic strengthening during spindles ( 989 ).
Spindles also appear to be involved in the consolidation of skills, especially of simple motor (e.g., pursuit rotor task, simple sequential finger tapping) and visuomotor skills ( 1114 ). Increases of spindles (13–15 Hz) and stage 2 sleep are particularly observed following training of tasks like figure tracing ( 59 , 394 – 396 , 399 , 837 , 920 , 921 , 1173 , 1174 ), which were also revealed to be vulnerable to stage 2 sleep deprivation ( 1112 , 1117 ). In some studies the increase in spindle activity was topographically restricted to the cortex areas most strongly involved in skill performance, i.e., in the contralateral motor cortex after motor tapping training ( 862 ) and in the parietal cortex after training in a visuospatial skill ( 214 ). Also, correlations between different parameters of spindle activity or stage 2 sleep and overnight improvements in motor skills have been consistently reported ( 562 , 862 , 1216 , 1286 , 1287 ), and these correlations were revealed for fast rather than slow spindles, in the studies distinguishing these two spindle types ( 59 , 962 , 1174 ).
In spite of the quite robust correlational evidence for the involvement of spindles in memory processing, a causal role of spindles in memory consolidation has not yet been demonstrated, as this requires the selective experimental manipulation of spindle activity. Approaches to increase spindle activity pharmacologically by administration of GABA A receptor agonistic drugs do not appear to be suitable in this regard, as they concurrently decrease SWA (see sect. V A2 ). In another approach, neurofeedback training to voluntarily increase EEG power in the 11.6–16 Hz sigma frequency band produced a small increase in sigma power also during subsequent sleep ( 86 ). This increase, however, was not associated with any change in the overnight retention of declarative memories.
D. Sharp Wave-Ripples
Hippocampal sharp waves are fast, depolarizing events generated in CA3 that become superimposed by ripple activity [i.e., high-frequency local field potential oscillations (100–300 Hz) originating in CA1] to form SW-R events ( 159 , 162 , 196 , 241 , 459 , 832 , 1342 ). SW-Rs occur mainly during SWS but also during nonexploratory wakefulness (e.g., drinking, grooming, and quiet wakefulness). Ripples arise from an interaction between inhibitory interneurons and pyramidal cells via synaptic (glutamatergic, GABAergic) connections and gap junctions ( 162 ). Hippocampal stimulation protocols that induce LTP concurrently facilitate the generation of SW-Rs in CA3, and SW-Rs during sleep can be initiated by neurons whose recurrent connectivity had been transiently potentiated during preceding wakefulness ( 70 ). SW-Rs may conversely promote LTP and spike time-dependent plasticity in hippocampal circuits ( 97 , 159 , 241 , 640 , 777 ). Modulation of neuronal connectivity during ripples might be specific to local circuits, because firing during single ripples involves only small subpopulations of pyramidal cells and is highly variable across multiple succeeding ripples ( 241 , 1342 ). Most importantly, SW-Rs during SWS typically accompany the reactivation of neuron ensembles active during the preceding wake experience (see sect. III A ).
In rats, learning of an odor-reward association task produced a strong and long-lasting (up to 2 h) increase in the magnitude of ripples and the number of ripple events during subsequent SWS ( 355 ). Similarly, after a spatial learning task, increases in ripple density during postlearning sleep were significantly correlated with the formation of associative spatial memories ( 955 ). In epileptic humans, the number of rhinal ripples during a nap correlated positively with the consolidation of previously acquired picture memories ( 50 ). Two recent studies in rats demonstrated a causal role of SW-Rs in memory consolidation ( 340 , 458 ). In both studies, emergent ripple events were selectively disrupted by electrical stimulation during the rest period after learning, without disturbing sleep, which distinctly impaired consolidation of the acquired spatial memories. In conclusion, there is now good evidence that SW-Rs are critically involved in the consolidation of hippocampus-dependent memories during sleep.
E. Slow Oscillations, Spindles, and SW-R Interact
The fine-tuned temporal relationship between the neocortical slow oscillations, thalamic spindles, and hippocampal SW-Rs originates from a top-down control of the slow oscillation on the two other events. In addition to the neural synchronization in the neocortex, the synchronizing effects of slow oscillations spread to thalamic and hippocampal as well as other brain regions involved in off-line memory consolidation. At the neocortical level, the slow oscillation in terms of EEG rhythms strongly modulates the amplitude of faster beta and gamma frequencies which is reduced to a minimum during the hyperpolarizing down-phase of the slow oscillation ( 224 , 240 , 828 , 829 ). Generation of spindles in the thalamus and generation of SW-Rs in the hippocampus are distinctly suppressed during the down-phase of the slow oscillation, and this is followed by a rebound in spindle and SW-R activity during the succeeding depolarizing up-state ( 66 , 215 , 597 , 829 – 832 , 925 , 1088 ). As for spindles, only the classic fast spindles display the strong phase synchronization with the emergent depolarizing up-state of the slow oscillation. Slow frontal spindles, in contrast, tend to follow fast spindles by 200–500 ms and thus occur already in the up-to-down state transition of the slow oscillation ( 38 , 826 ). As for the modulation of SW-Rs, this appears to be entirely driven by cortical inputs, as the hippocampus itself does not generate slow oscillations ( 597 ). However, membrane potentials with a slight delay (of ∼50 ms) follow the up- and down-states of neocortical slow oscillations, particularly in dentate gyrus and CA1 ( 502 , 503 , 1333 ). In parallel, neocortical up states might time-lock spontaneous activity in the medial entorhinal cortex, a major gateway between the neocortex and hippocampus ( 501 ). There is also evidence for a slow oscillation modulation of locus coeruleus burst activity with preferential locus coeruleus firing during the down-to-up state transition of the slow oscillation ( 353 , 727 ).
Importantly, prior learning appears to strengthen the top-down control of slow oscillations on spindles and ripples. In humans, intense learning of vocabulary did not only increase the slope of the down-to-up state transition of the slow oscillation during succeeding non-REM sleep, but concurrently enhanced fast spindle activity, with this increase concentrating on the up-states of the slow oscillation, whereas no changes were observed in hyperpolarizing down-states ( 826 , 829 ). Interestingly, these analyses also revealed that learning promoted the occurrence of trains of several succeeding slow oscillations. In these trains, fast spindles were not only driven by the depolarizing slow oscillation up-state but appeared to feed themselves back to enforce the succeeding slow oscillation, as well as the likelihood of associated slow frontal spindles. The enhancement of such slow oscillation-spindle cycles might be a key mechanism whereby fast spindles initiate the consolidation of newly learned materials during sleep ( 826 ). Learning also increases hippocampal SW-Rs ( 355 , 955 ), and it is likely that learning-induced increases in slow oscillations strengthen parallel to the synchronization of enhanced SW-R activity and the depolarizing up-state of the slow oscillation, which remains to be demonstrated.
SW-Rs are also temporally coupled to spindles ( 1075 , 1088 ) which can only be partly explained by the common driving impact of the slow oscillation on both phenomena ( 829 ). Event-correlation histograms derived from intracranial recordings in rat and humans revealed that ripples are associated with a rise in spindle activity that starts shortly before ripple onset and then outlasts the ripple ( 215 , 216 , 829 , 832 ). The rise in spindle activity was even more persistent (up to 2 s) when the rats had performed on a learning task prior to sleep ( 829 ). Moreover, fine-grained temporal analyses revealed that the co-occurrence of spindles and ripples leads to the formation of so-called “spindle-ripple events” where individual ripple events become temporally nested into succeeding troughs of a spindle ( 216 , 1075 , 1317 ). As ripples accompany assemble reactivations in the hippocampus, spindle-ripple events might represent a mechanism serving the sequenced transfer of reactivated memory information towards neocortical sites ( 827 , 1087 ). Importantly, the formation of spindle-ripple events is restricted to classic fast spindles, which (like SW-Rs) are driven by the depolarizing down-to-up state transition of the slow oscillation. In contrast, the slow frontal spindles typically occur 200–500 ms later in the slow oscillation cycle (i.e., at the up-to-down state transition), and thus also tend to follow hippocampal SW-Rs with the same delay ( 216 ). In contrast to fast spindles, the neocortex might be “functionally deafferented” from its hippocampal inputs during frontal slow spindles ( 925 ). Taken together, these data suggest a looplike scenario during sleep after learning. While thalamo-cortical sleep spindles enforce the generation of hippocampal ripples, ripple events in turn feed back to sustain the ongoing generation of spindle activity ( 828 ), and possibly also slow oscillation activity, although this is presently unclear ( 635 , 1087 ). Such looplike coordination goes along with an enhanced formation of spindle-ripple events whereby ripples (together with the reactivated hippocampal memory information they carry) are fed exactly into the excitatory phases of the spindle cycle. By still reaching neocortical networks during the depolarizing up-phase of the slow oscillation, the spindle-ripple event may thus serve as an effective mechanism for transferring hippocampal memory information towards neocortical long-term stores. Indeed, fast spindles do not only phase-lock hippocampal ripples but also neocortical gamma-band activity as an indicator of coherent information processing in local neocortical networks ( 52 ). Spindle-gamma coupling might be linked to facilitate synaptic plastic processes underlying the storage of information in neocortical circuitry ( 1012 ).
In sum, there is now growing evidence for a dialogue between neocortex and hippocampus mediating the system consolidation of hippocampus-dependent memory, which is orchestrated by a fine-tuned interaction between oscillatory field potential activities. In this dialogue, the neocortical slow oscillation provides a top-down temporal frame that synchronizes the reactivation of hippocampal memories with the simultaneous occurrence of fast spindles to enable the formation of spindle-ripple events. Spindle-ripple events occurring during the slow oscillation up-states, conversely, provide a bottom-up mechanism for the transfer of reactivated memory information from the hippocampus to the neocortex where they might effectively support plastic synaptic processes underlying the storage of this information.
F. PGO Waves and Theta-Rhythm of REM Sleep
1. pgo waves.
PGO waves are driven by an intense burst of synchronized activity that propagates from the pontine tegmentum to the lateral geniculate nucleus and visual cortex. They occur in temporal association with rapid eye movements in rats and cats. They are not readily identifiable in the human EEG, though fMRI studies revealed activations in pontine tegmentum, thalamus, primary visual cortex, putamen, and limbic areas associated with rapid eye movements during REM sleep, which might be linked to PGO waves ( 824 , 1312 ). PGO waves tend to occur phase-locked to theta oscillations ( 618 ). Like theta activity, PGO waves have been proposed as a mechanism supporting synaptic plasticity in the regions they reach ( 258 , 264 ). Training on an active avoidance task is followed by a robust increase in PGO wave density for 3–4 h after training, and changes in PGO wave density were also proportional to the improvement in task performance between initial training and post-sleep retest ( 259 , 261 , 1225 ). Moreover, PGO wave density during posttraining REM sleep is correlated with increased activity of plasticity-related immediate early genes and brain-derived neurotrophic factors in the dorsal hippocampus. These increases were abolished after selective elimination of the PGO wave generating cells in the brain stem and enhanced after cholinergic stimulation of these cells ( 261 , 1225 ).
2. Theta activity
Theta activity, i.e., periods of synchronized activity in the 4–8 Hz frequency band, is a hallmark of tonic REM sleep (REM sleep without actual rapid eye movements) ( 161 ) and correlates with rapid eye movements and PGO waves ( 618 – 620 ). Primary generating mechanisms appear to be located within the hippocampus, i.e., in CA1 ( 472 ), although extrahippocampal input, mainly from the septum, contributes. In rodents, theta predominates in the hippocampus and associated areas where it is likewise seen during awake exploratory behaviors. In humans, EEG theta activity during REM sleep is less coherent and persistent in hippocampal regions and is seen as well in neocortical areas, again also during wakefulness ( 174 , 860 , 1222 , 1223 ). Theta activity in waking is considered a condition favoring the encoding of new information and associated synaptic plastic processes in hippocampal networks ( 64 , 371 , 612 , 1251 ). Burst stimulation of CA1 inputs can induce LTP or LTD depending on whether it arrives at the peak or trough, respectively, of ongoing theta oscillations ( 561 , 892 ). Likewise, LTP and LTD, respectively, can result from slight differences in the phase of theta oscillations between pre- and postsynaptic neurons ( 514 ). Theta activity also modulates the amplitude of high-frequency gamma oscillations (∼40 Hz), i.e., a rhythm likewise thought to favor neuronal encoding and spike time-dependent plastic processes ( 173 , 242 , 420 ), with the theta-phase coupling of such faster oscillations differing between phasic and tonic REM periods ( 133 ). Phase-locking of gamma band activity during coherent theta activity in prefrontal-hippocampal circuitry is thought to underlie the successful (explicit) encoding of hippocampus-dependent memory information during wakefulness ( 72 , 74 , 220 , 423 ).
Evidence for an involvement of theta activity during REM sleep in memory consolidation is overall meager. Rats showed increased theta activity during REM sleep after training on an avoidance task ( 397 ). However, after fear conditioning, mice exhibited reduced REM sleep theta ( 529 ). Interindividual variability in fear learning across sleep was related to bidirectional changes in theta coherence between the amygdala, medial frontal cortex, and the hippocampus during REM sleep ( 941 ). In the two studies reporting signs of firing pattern reactivation in hippocampal neuron assemblies during REM sleep after performance on spatial tasks, these reactivations were specifically linked to ongoing theta activity, with reactivations expressing either during a specific phase of the theta cycle or linked to a specific amplitude modulation of ongoing theta activity ( 744 , 935 ) (see sect. III A3 ). In humans, scalp-recorded EEG theta activity was enhanced during REM sleep following learning of paired associates ( 399 ) and was correlated with consolidation of emotional memories (specifically over the right prefrontal cortex relative to the left) ( 861 ). However, the overnight reduction in amygdala activation in response to emotional pictures presented before and after sleep was correlated with gamma rather than theta activity during intervening REM sleep ( 1231 ). In patients with Alzheimer's disease, theta activity during not only REM but also SWS was faster compared with age-matched controls, and fast theta activity correlated with better overnight memory formation ( 572 ).
While these data so far do not speak for any essential function of REM sleep theta activity in memory consolidation, the characteristics of theta activity as well as faster EEG frequencies during REM sleep point out an important feature relevant to putative memory processing during this sleep stage. Compared with wakefulness or SWS, EEG activity during REM sleep shows reduced coherence between limbic-hippocampal and neocortical circuitry in a wide range of frequencies including theta and gamma ( 51 , 174 , 293 ). Similarly, within hippocampal circuitry, gamma band activity shows reduced coherence across the CA3 and CA1 regions during tonic REM sleep compared with activity during wake exploration ( 834 ), suggesting altogether diminished coordinated information flow between hippocampal input and output regions and between the hippocampus and neocortex during tonic REM sleep. At the same time, local information processing might be enhanced, as evidenced by increased theta and gamma synchrony between dentate and CA3 to levels even higher than during wakefulness, as well as by the general high levels of theta and fast EEG frequencies in hippocampus and neocortex during REM sleep. It has been argued that such high levels of local information processing in the absence of coordinate long-range communication, together with the specific neurochemical milieu during REM sleep, represent conditions that favor processes of synaptic consolidation ( 293 ). In fact, a recent study showed that brain activation during intervening REM sleep periods might contribute to an overall downscaling of neuronal firing rates observed across sleep ( 485 ). While discharges in hippocampal CA1 neurons increased during single non-REM episodes, firing rates decreased from pre-REM non-REM periods to post-REM non-REM periods, with the decrease being correlated with theta power during intervening REM sleep. Interestingly, the general decrease in firing rate during REM sleep was accompanied by an increase in firing synchrony and a decrease in the variability of cell firing specifically during ripple events. Specifically, across non-REM-REM-non-REM triplets, the discharge rate of hippocampal neurons decreased between ripple events and increased during ripple events, and this increase in synchrony was likewise correlated with theta power during intervening REM sleep. These data suggest that REM sleep theta is not only involved in an unspecific synaptic downscaling but also in reorganizing and shaping hippocampal memory representations ( 118 ).
V. NEUROCHEMICAL SIGNALING AND MEMORY CONSOLIDATION DURING SLEEP
Sleep and sleep stages are characterized by a specific neurochemical milieu of neurotransmitters and hormones (see FIGURE 1 C ), some of which contribute to memory consolidation by favoring processes of synaptic consolidation (i.e., synaptic LTP or synaptic LTD and depotentiation) or processes of system consolidation. Neurochemical studies of sleep-associated plasticity have taken two distinct approaches: 1 ) they have examined signals known to be essential for synaptic plasticity, such as glutamatergic synaptic transmission and the cascade of intracellular signaling mediating LTP, LTD, as well as changes in synaptic morphology thought to underlie long-term memory; or 2 ) they have examined signals known to be essentially involved in the regulation of sleep.
A. LTP/LTD During Sleep
Synaptic LTP and LTD are considered basic neurophysiological mechanisms underlying the formation of memory. LTP and LTD have been mainly studied in excitatory glutamatergic synapses but occur also in inhibitory GABAergic synapses. In glutamatergic synapses, LTP is induced via activation of postsynaptic NMDA receptors and subsequent calcium (Ca 2+ ) influx, which leads to activation of calcium-sensitive kinases like Ca 2+ /calmodulin kinase II (CaMKII) and protein kinase C (PKC) that in turn activate transcription factors and immediate early genes that can eventually lead to an altered protein synthesis and resculpturing of synapses ( 4 , 983 ). An early and late phase of LTP and LTD is discriminated with only late LTP (>3 h) requiring new protein synthesis. Maintenance of LTP, i.e., the development of late LTP from early LTP, is assumed to require a “tagging” of synapses due to associative heterosynaptic stimulation within a certain time window (∼30 min) following LTP induction ( 419 ). Heterosynaptic tagging can involve noradrenergic and dopaminergic transmission, but also the activation of mineralocorticoid receptors. The expression of BDNF and PKM-ζ are further important signals not only supporting induction of LTP but in particular for mediating the emergence of late LTP ( 746 , 850 , 1020 ).
It is likely that memory consolidation during sleep involves both synaptic and system consolidation processes ( 1305 ) (see sect. I B ). Sleep might enhance memory by directly favoring late LTP and LTD in synaptic networks that were potentiated during the preceding wake phase. Alternatively, this form of synaptic consolidation may occur as part of a system consolidation process in which newly encoded memories are reactivated and redistributed to other networks during sleep where they subsequently undergo synaptic consolidation (see sect. II F ). Considering that reactivation and redistribution of memory representations constitute a central mechanism of memory consolidation during sleep, then the basic question arises whether these reactivations during sleep can newly induce LTP and LTD or merely serve to support the maintenance of LTP and LTD induced during prior waking. These issues are presently far from being clear. Nevertheless, investigations of sleep-associated changes in the molecular signals that mediate the induction and maintenance of LTP and LTD have provided some important clues about possible contributions of sleep to persisting plastic synaptic changes. As discussed in section IV B , several studies show an increase in extracellular glutamate concentrations as well as molecular markers of LTP across wake periods and a decrease after sleep periods, particularly after SWS ( 204 , 205 , 207 , 257 , 457 , 607 , 940 , 1264 ), suggesting that the induction of new LTP is more likely during wakefulness than sleep. While experimental induction of LTP and LTD is feasible during wakefulness and REM sleep, induction of long-lasting changes was less likely (although not impossible) during SWS ( 130 ). Sleep might, nonetheless, be critically involved in processes supporting the long-term maintenance of synaptic changes, particularly in those involving protein synthesis. More importantly, most of these studies assessed expression of the relevant signals on a global scale in large regions of the brain and cortex, and the extent of encoding of information, i.e., learning during wakefulness before sleep, was not systematically varied ( 989 ). Hence, this approach leaves open the basic question of how sleep specifically affects newly formed memory traces and underlying synaptic plasticity in discrete neuronal circuits.
1. Glutamate and intracellular PKA signaling
Blocking of ionotropic glutamate receptors during postlearning sleep was used as a pharmacological tool to study whether sleep-dependent memory consolidation involves the reactivation of glutamatergic synapses. Consistent with this hypothesis, in humans, blocking of NMDA or AMPA receptors by infusion of ketamine or caroverine during post-learning retention sleep completely abolished overnight gains on a visual texture discrimination task ( 435 ), presumably mediated by local synaptic plastic changes in the visual cortex ( 1049 , 1051 ). Surprisingly, ketamine or caroverine administration during retention sleep did not impair consolidation of hippocampus-dependent declarative memories (word pairs) (unpublished observation). However, in these studies consolidation of word pair memories during sleep distinctly benefited from administration of d -cycloserine, a partial agonist of the NMDA receptor that acts at the glycine binding site to enhance Ca 2+ influx. These data suggest that the consolidation of hippocampus-dependent memory involves the reactivation of glutamatergic synapses, although the exact mechanisms of glutamatergic reactivation might differ between memories with primary hippocampal and primary neocortical representations.
In animals, sleep-dependent plastic changes were also revealed to critically depend on glutamatergic and LTP-related mechanisms ( 411 , 1066 ). In the developing cortex of cats, ocular dominance plasticity induced by monocular deprivation of one eye requires sleep ( 77 , 412 , 413 ). These sleep-dependent changes are prevented by blocking NMDA receptors or cAMP-dependent protein kinase (PKA) during sleep after monocular experience ( 48 ) (see sect. VII A3 ). Blockade of NMDA and PKA signaling was associated with reduced activation of the kinases CaMKII and ERK as well as phosporylation of GluR1 at Ser831, i.e., processes that are critical to the insertion of AMPA receptors into the postsynaptic membrane and the strengthening and maintenance of LTP. In mice, treatment with the selective phosphodiesterases (PDE) 4 inhibitor rolipram immediately and 2.5 h after single-trial contextual fear conditioning prevented the impairing effect of sleep deprivation on retention of the freezing response to the conditioned context ( 1246 ). These observations corroborate the concept that impaired memory retention following sleep deprivation results from the disruption of the cAMP-PKA-CREB pathway that supports the formation of late LTP. Impaired signaling in this pathway originates from increases in PDE activity during sleep deprivation, with rolipram counteracting this process ( 477 , 498 – 500 , 546 , 1246 ). Based on this research, Abel and colleagues ( 546 ) suggested that cAMP-PKA dependent consolidation in the hippocampus occurs as a “sort of molecular replay” of the same molecular events recruited during prior encoding of information during wakefulness. This notion fits well to a model proposed earlier by Ribeiro and Nicolelis ( 988 ), suggesting that memory encoding during waking involves calcium-dependent genetic processes which trigger plasticity-related gene regulation during subsequent sleep. Similar ideas have been formulated by Benington and Frank ( 77 ) as well Datta and colleagues ( 261 ). The molecular replay may mainly occur during REM sleep enabled through coactivation of muscarinic receptors in the presence of high global cholinergic and low serotonergic activity, although it may similarly occur in local circuitry during non-REM sleep ( 475 , 546 ).
Also, cAMP-PKA-dependent memory formation for contextual fear conditioning is selectively sensitive to the inhibition of PKA and protein synthesis when this inhibition occurs within specific time windows after training, i.e., immediately or 4 h after training ( 128 ). Correspondingly, specific time windows of increased REM sleep and sensitivity to REM sleep deprivation have been observed after learning of hippocampus-dependent tasks (Morris water maze with hidden platform, radial maze) ( 1115 ), and blockade of NMDA receptors shortly after these REM sleep windows disrupted sleep-dependent consolidation of these tasks ( 1094 , 1099 ) (see sect. II B ).
GABAergic inhibition via interneurons is embedded in almost all central nervous networks and contributes essentially to both the induction of sleep via brain stem and hypothalamic mechanisms, and the local control of LTP and LTD at glutamatergic synapses. GABAergic agonists, like benzodiazepines that are also used clinically to improve sleep, were consistently revealed to impair LTP as well as LTP-related plasticity in glutamatergic synapses via activation of the ionotropic GABA A receptor (e.g., Refs. 225 , 277 , 549 , 575 , 633 , 1008 , 1270 ). In addition, GABAergic synapses themselves undergo plastic changes that predominate during early development, but some of which can be observed in the mature brain as well ( 683 ). Yet, it is presently unclear how these processes contribute to hippocampal memory formation.
In humans and rats, systemic administration of agonistic modulators of the GABA A receptor, including barbiturates, benzodiazepines, and zolipedem, reduces sleep latency and consistently increases both sleep in non-REM stage 2 and sleep spindles, but typically simultaneously reduces SWS and SWA ( 684 ). This pattern diverges from the concordant increase in both spindles and SWA typically associated with memory processing during sleep following intense learning. Interestingly, nonspecifically enhancing availability of GABA in the synaptic cleft by administration of the GABA reuptake inhibitor tiagabin induced profound increases in SWS and SWA paralleled by marked declines in light sleep and REM sleep in both rats and humans, leaving spindle activity unaffected ( 686 , 793 , 1299 , 1301 ). Selective GABA A or GABA B receptor agonists like gaboxadol, muscimol, or γ-hydroxybutyrate produced similarly robust increases in SWS and SWA, while effects on spindle activity differed between drugs ( 304 , 684 , 685 , 702 , 1228 , 1297 , 1298 , 1300 ). At the network level, GABA A agonistic drugs like diazepam in slice preparations suppressed the generation of SW-Rs that are known to accompany the neuronal reactivation of newly encoded memory representations during SWS. The effect was dose-dependent and not observed following the GABA A -receptor modulator zolpidem ( 655 ). Conversely, the GABA A agonist muscimol and also zolpidem impaired sleep-dependent plastic changes in the visual cortex in a developmental model of synaptic plasticity in cats [i.e., ocular dominance plasticity (ODP); see sect. VII A3 for details] ( 48 , 411 , 1059 ), suggesting that GABA plays a critical role for plastic processes occurring during sleep.
GABAergic effects on sleep-dependent consolidation of memory in humans have not been thoroughly investigated thus far, which is surprising given that GABA-agonistic substances like benzodiazepines are widely used for the treatment of sleep disorders. Interestingly, there is rather consistent evidence that benzodiazepines administered after the encoding of information can enhance memory for this information in waking subjects, possibly by preventing the encoding of new interfering information after learning ( 247 , 888 , 1313 , 1332 ). However, the few studies concentrating on memory consolidation during sleep point towards opposite effects. Early experiments in healthy volunteers suggested an impairing influence of the benzodiazepine trialzolam and the non-benzodiazepine zopiclone administered after learning before sleep on the retention of words ( 1040 , 1082 ), whereas one study did not find an impairing effect of the hypnotics zolpidem and triazolam on sleep-dependent memory consolidation of words and nonwords ( 813 ). Administration of the GABA B agonists sodium oxybate or baclofen before a nap increased SWS, without affecting sleep-dependent declarative memory consolidation of word pairs and face-location associations ( 1255 ). In rats, administration of the GABA A agonists eszopiclone and zolipem after learning before sleep impaired contextual memory tested 24 h later ( 577 ). Two more recent studies in humans showed similarly impairing effects of triazolam and zolpidem on sleep-dependent consolidation of finger sequence tapping skills ( 835 , 836 ). This is remarkable, as the impairment in motor skill consolidation was paralleled by robust increases in the amount of non-REM sleep stage 2 and spindle activity as well as REM sleep in these studies. Sleep-dependent consolidation of motor skill memories was also impaired following administration of the GABA reuptake inhibitor tiagabin, and this impairment was paralleled by a substantial increase in SWS and SWA ( 370 ). No effects on declarative memory consolidation (word pairs) were found in this study. The negative findings after benzodiazepine or tiagabin administration during postlearning sleep suggest that EEG phenomena-like spindles and SWA do not represent mechanisms that per se are sufficient to support sleep-dependent memory consolidation. Yet it is not clear whether pharmacologically induced spindles and SWA are functionally equivalent to their endogenous counterparts.
B. Neurotransmitters Involved in Sleep Regulation
Most neurotransmitters and neuromodulators involved in sleep regulation are also involved in processes of memory and plasticity, speaking in favor of a high degree of overlap and functional interaction between these processes. The regulation of sleep and wakefulness is based on a balanced interaction between wake-promoting networks centered in the upper brain stem and lateral hypothalamus and sleep-promoting networks in the anterior hypothalamus, which mutually inhibit each other to enable transitions into the respective brain states depending on a switchlike mechanism ( 146 , 262 , 1032 ). The wake-promoting network includes mainly cholinergic neurons of peduncolopontine and laterodorsal tegemental nuclei (PPT, LDT), the noradrenergic locus coeruleus (LC), and the serotonergic dorsal and median raphe nucleus, as well as histaminergic neurons in the hypothalamic tuberomammillary nucleus (TMN), which have widespread projections to the lateral hypothalamus, basal forebrain, and cerebral cortex. Activity in this network is reinforced by orexin A and B (hypocretin 1 and 2) producing neurons in the posterior lateral hypothalamus (adjacent to the TMN). The sleep-promoting network includes mainly the ventrolateral (VLPO) and median (MnPO) preoptic nuclei of the hypothalamus, which contain neurons releasing GABA and galanin to inhibit the wake-promoting network at all levels.
The sleep-promoting network is stimulated by neurons sensing astrocytic adenosine that accumulates extracellularly as a rundown product of cellular metabolism at least in some parts of the brain ( 78 , 506 , 942 , 1160 ). Signaling of adenosine via A1 receptors, which are diffusely distributed in the brain, may directly inhibit neurons of the wake-promoting arousal system (e.g., Refs. 741 , 867 , 884 ). A2a receptors located close to the VLPO mediate a direct sleep-inducing effect that is counteracted by A2a-receptor blockers like caffeine ( 578 , 1035 ).
Transitions between non-REM and REM sleep are mediated by a balanced interaction in brainstem pontine networks between GABAergic neurons in the sublaterodorsal region (precoeruleus) that fire during REM sleep (REM-on) and GABAergic neurons in the periaqueductal gray matter and the adjacent lateral pontine tegmentum (vlPAG, LPT) that fire during Non-REM sleep to inhibit the REM-on neurons. This core REM switch is in turn modulated by noradrenergic LC neurons and serotonergic neurons of the dorsal raphe nucleus that act on both sides of the switch to inhibit REM sleep and cholinergic neurons of the PPT and LDT promoting REM sleep. Via the same core REM switch, hypothalamic orexin neurons inhibit, whereas VLPO neurons promote REM sleep ( 1032 ).
As a result of this regulation of non-REM and REM sleep, widespread changes in activity occur also for the wake promoting neuromodulators, mainly activity of acetylcholine, norepinephrine, and serotonin. Cholinergic activity reaches a minimum during SWS, whereas during REM sleep it reaches levels well comparable or even higher compared with cholinergic activity during wakefulness. Noradrenergic and serotonergic activity reaches a minimum during REM sleep, and is at an intermediate level during SWS. At the neurohormonal level, early periods of SWS are associated with a strong activation of somatotropic activity, whereas late periods of REM sleep are characterized by an increased release of corticosteroids from the adrenals.
1. Effects of wake- versus sleep-promoting signals on LTP and LTD
Wake-promoting signals have been mainly found to support LTP induction and maintenance. The wake-promoting neuropeptide orexin robustly facilitated LTP induction in the ventral tegmental area (VTA) and in the hippocampus, directly and via LC noradrenergic release, respectively ( 115 , 1296 ). Supporting effects on the induction and maintenance of LTP were also consistently revealed for histamine ( 677 , 750 ), norepinephrine, as well as acetylcholine ( 140 , 585 , 598 , 1187 , 1309 ). Indeed, norepinephrine and acetylcholine are also important for the induction of late LTP ( 12 , 1295 ) and were often found to synergistically act to enhance LTP ( 140 , 1309 ) as well as LTD ( 642 ). Serotonergic activity displaying sleep-dependent fluctuations similar to those of noradrenergic activity appears to influence LTP and LTD in a less homogeneous way, with its effects strongly depending on the brain region, receptor subtype activated, and the type of stimulation used to induce LTP or LTD ( 98 , 576 , 601 , 628 , 654 , 675 ).
The major sleep-promoting factor adenosine was also revealed to contribute to the regulation of synaptic plasticity. Via activation of A1 receptors extracellular adenosine attenuates hippocampal LTP, whereas A2A receptor activation mediates an enhancing effect ( 233 , 816 , 972 ). A2A receptor activation is also critically involved in the enhancing effects of BDNF on hippocampal LTP ( 402 ). On the other hand, LTP as well as basal excitatory synaptic transmission in orexin neurons of the lateral hypothalamus can be diminished by activation of adenosine A1 receptors ( 1339 ). It has been proposed that under basal firing conditions, glia-derived extracellular adenosine mainly activates A1 receptors leading to a diffuse inhibition of synaptic transmission, whereas with high-frequency synaptic stimulation A2A receptors are activated by adenosine which is formed locally by ecto-nucleotidases from synaptically released ATP and overrides A1 receptor-mediated effects ( 245 ). LTP induced at hippocampal Schaffer collateral synapses can be reversed by low-frequency (1–2 Hz) stimulation mimicking basal conditions as they could occur during SWS, and this depotentiation appears to be essentially mediated through diffuse activation of A1 receptors ( 576 ). The depotentiation triggered in this way by direct neocortical inputs might represent a mechanism helping to reset synaptic transmission in the hippocampus, thus preparing these networks for further encoding of information ( 599 ).
2. Neuromodulation associated with sleep and SWS, and memory processing
The neuromodulatory changes characterizing SWS are indeed the same that accompany the induction of sleep. Studies specifically targeting the role of neurochemical conditions during SWS for memory processing have so far concentrated on astrocytic adenosine signaling, the minimum levels of cholinergic activity and intermediate-level, pulsatile activity of noradrenergic systems during SWS, as well as on SWS-related changes in hormonal systems.
Regarding astrocytic adenosine, Halassa and co-workers ( 393 , 506 ) showed that genetic inhibition of gliatransmission in mice attenuates the accumulation of sleep pressure as indicated by decreased sleep time, decreased duration of non-REM sleep bouts, and decreased SWA activity in response to sleep deprivation. Despite decreased SWA, these mice, unlike wild-type mice, did not exhibit signs of impaired recognition memory on an object recognition task when they were sleep deprived after training. Infusion of the adenosine A1 receptor antagonist 8-cyclopentyl-1,3- dimethylxanthine (CPT) suppressed sleep only in the wild-type mice and mimicked the transgenic phenotype with regard to both sleep and memory effects. Parallel effects were demonstrated for the sleep deprivation-induced impairment of hippocampal late-phase LTP. Recently, Schmitt and colleagues ( 1047 ) showed that the level of the adenosine A1 receptor activation increases during normal and prolonged wakefulness in mice, and that this increase affects synaptic transmission in the hippocampus as well as network activity in the cortex. These effects were prevented by genetic inhibition of gliatransmission. Taken together, these findings indicate that astrocytic adenosine, beyond its promoting effect on sleep, via activation of A1 receptors is also involved in mediating the impairing effects of sleep deprivation on memory consolidation.
Suppression of cholinergic activity during SWS alleviates tonic inhibition of hippocampal CA3 and CA1 feedback neurons, thereby it enables spontaneous reactivations of the hippocampal networks and of the memory information encoded in these networks, as well as the transfer of the reactivated information to neocortical networks ( FIGURE 6 A ; Refs. 520 , 522 , 782 ). Consistent with this concept of low acetylcholine enabling systems consolidation of declarative memories, increasing cholinergic tone during a period of SWS-rich sleep by administration of the cholinesterase inhibitor physostigmine completely blocked the sleep-associated consolidation of word pair memories ( FIGURE 6 B ; Refs. 429 , 963 ). Some subjects showed slight decreases in SWA after physostigmine, but these were unrelated to the impairment in declarative memory consolidation, suggesting that cholinesterase inhibition primarily affected memory processing in hippocampal rather than thalamo-cortical circuitry. Conversely, blocking cholinergic receptors in waking subjects by simultaneous administration of nicotinic and muscarinic receptor antagonists improved the consolidation of declarative memories during wakefulness, but concomitantly decreased the ability to encode new information ( 963 ). These results strongly support the notion that high acetylcholine levels are critical for successful encoding, whereas low acetylcholine levels facilitate consolidation of memories, suggesting that acetylcholine might function as a switch between brain modes of encoding and consolidation as established during waking and SWS, respectively ( 522 ). Manipulating solely nicotinic or muscarinic receptor activity remained ineffective in these studies ( 864 , 963 ). Since the cholinergic neurons mediating the recurrent inhibition in hippocampus receive strong inhibitory GABAergic inputs, strengthening this GABAergic activity should enhance hippocampal memory reactivation. This mechanism could explain why postlearning administration of benzodiazepines improves memory consolidation in waking subjects but not during SWS when cholinergic activity is already minimal ( 454 , 813 ).

Influence of cholinergic activity on memory consolidation during wakefulness and sleep. A : concept: during active waking, acetylcholine (ACh) levels are high. Information encoded by neocortical structures flows through the entorhinal cortex and dentate gyrus (DG) into hippocampal region CA3 (connections less sensitive to modulation by ACh; thick arrows). Connections suppressed by ACh modulation (dashed arrows) to region CA1, entorhinal cortex, and association cortex are strong enough to mediate immediate retrieval, but do not overwhelm the feed-forward connectivity, ensuring efficient encoding. In contrast, during SWS, ACh levels are low, and memories are reactivated in region CA3 during sharp wave-ripples (SW-Rs). These waves of activity flow back through region CA1 to entorhinal cortex and neocortex, enabling an efficient redistribution of memory representation (system consolidation) underlying long term memory storage. [Adapted from Hasselmo ( 520 ), with permission from Elsevier.] B : in accordance with the model, increasing cholinergic tone in humans by administration of the acetylcholineesterase inhibitor physostigmine during postlearning SWS impairs consolidation of declarative memory (word pairs) during sleep, compared with placebo. In contrast, combined blockade of muscarinic and nicotinic cholinergic receptors during a postlearning wake interval (by administration of scopolamine and mecamylamine) enhanced consolidation of declarative memory (word pairs) during this wake interval. Simultaneously, the combined receptor blockade impaired new encoding (of numbers). Values are means ± SE: * P ≤ 0.05; ** P ≤ 0.01. [Data from Gais and Born ( 429 ) and Rasch et al. ( 963 ).]
Noradrenergic activity during SWS, arising from the LC as the brain's main source of norepinephrine, seems to be particularly related to the depolarizing up-state of the slow oscillations as LC firing has been found to be entrained to these up-states ( 353 ). Moreover, a specific sleep-related window of increased LC burst activity ∼120 min after learning of odor-reward associations has been identified in rats ( 356 ). In humans, suppressing noradrenergic LC output by administration of the alpha2-autoreceptor agonist clonidine during a SWS-rich retention period reduced consolidation of odor memories, whereas the retention of these memories was enhanced when availability of epinephrine during sleep was increased ( 434 ). In a subsequent study, clonidine infused during a SWS-rich period of retention sleep appeared to impair specifically emotional memory processing ( 1031 ). Whereas temporal order of emotional stories was better remembered compared with neutral stories in the placebo condition, clonidine blocked this superiority of emotional memory consolidation during SWS-rich sleep. Together, these findings suggest that bursts of noradrenergic activity during SWS are particularly important for the consolidation of memories that involve both a strong amygdala-mediated emotional component as well as a hippocampus-mediated declarative component, as keeping temporal order in the events of an episode represents a key function of the hippocampal formation (e.g., Refs. 285 , 722 , 761 ). Phasic burst of noradrenergic LC activity can enforce plasticity-related immediate early gene activity ( 207 , 208 ), and thereby contribute to LTP maintenance in circuitries that were potentiated during prior encoding. In the case of odor and emotional memories, these representations may partly reside in the basolateral amygdala as a main target of noradrenergic influences ( 804 ).
3. Hormonal modulation associated with sleep and SWS, and memory processing
SWS is associated with the inhibition of glucocorticoid release from the hypothalamus-pituitary-adrenal (HPA) system and a profound surge in the release of growth hormone releasing hormone (GHRH) and GH from the somatotropic axis ( 122 , 123 , 1271 ). While activation of the latter system might support hippocampal memory processing through brain-borne GHRH ( 430 , 509 ), inhibition of the former system affects limbic regions mainly via cortisol feedback that is downregulated during SWS. Low cortisol concentrations during SWS-rich sleep benefit declarative memory consolidation by preventing activation of glucocorticoid receptors that mediate an inhibitory influence on hippocampal LTP and output from CA1 ( 648 , 649 , 666 ). Accordingly, increasing glucocorticoid levels during sleep by postlearning administration of cortisol or dexamethasone impaired consolidation of memory for word pairs and for the temporal order in stories ( 932 , 933 , 1323 ). In addition, spontaneously increased nocturnal cortisol levels around midnight were found to be correlated with impaired retrieval of declarative memories in healthy subjects and patients with primary insomnia ( 58 ). However, a certain basal release of cortisol is necessary during SWS to sufficiently occupy mineralocorticoid receptors, which bind cortisol with a distinctly higher affinity and support the transition of early into late LTP in hippocampus. Accordingly, lowering cortisol levels in humans during early nocturnal SWS below baseline levels by pharmacological blockade of cortisol synthesis impaired sleep-dependent declarative memory consolidation ( 1272 ).
Melatonin is a circadian hormone that is released by the pineal gland during the night and, in diurnal animals and humans, substantially contributes to the entrainment of sleep to the night-time phase ( 212 ). Melatonin inhibits hippocampal LTP ( 223 , 877 , 1171 , 1307 ) and has also been found to impair acquisition of hippocampus-dependent spatial memories in rats ( 372 ) and of an active avoidance task in diurnal zebrafish ( 971 ), although divergent findings were obtained in humans ( 997 ). Whereas these observations point to an impairing influence of melatonin on the encoding of memory, there is preliminary evidence from fMRI experiments in humans that melatonin might enhance processes of hippocampal memory consolidation during sleep: parahippocampal activity patterns during retrieval of word-pair memories were found following a nap showed some similarity with those obtained when napping was replaced with administration of melatonin ( 471 ).
4. Neuro- and hormonal modulation associated with REM sleep, and memory
Cholinergic activity during REM sleep is high and comparable with that during waking, which might be particularly relevant for the consolidation of procedural memories during this sleep stage. Blocking muscarinic cholinergic receptors during “REM sleep windows” (i.e., time periods after learning in which REM sleep deprivation effectively impaired consolidation of respective materials, see sect. II B ) by administration of scopolamine consistently impaired memory in a habit learning version of the radial arm maze ( 720 , 721 ). Infusion of scopolamine into the dorsal striatum, a structure specifically involved in habit and skill learning, was particularly effective. In humans, the combined blockade of nicotinic and muscarinic receptors during REM-rich late sleep impaired off-line consolidation of a motor skill (finger sequence tapping) without affecting declarative memories ( 961 ). Conversely, increasing the availability of acetylcholine during post-training sleep by administration of an acetylcholinesterase inhibitor enhanced sleep-related benefits in a skill learning task ( 570 ). Anticholinergic treatment during wakefulness after learning remained ineffective ( 961 ). Taken together, these results identify high cholinergic tone as an important factor contributing to the off-line consolidation of procedural skills during REM sleep, in combination with other unknown processes. The effects are consistent with a role of acetylcholine in synaptic consolidation, e.g., by promoting activity of plasticity-related immediate early genes ( 475 , 615 , 1182 ) and maintenance of LTP in cortico-striatal networks ( 742 ).
There is some evidence that the increase in cholinergic activity during REM sleep is accompanied by an increased dopaminergic activity originating from ventral tegmental, rather than striatal, areas which innervate a network of further regions such as the nucleus accumbens and medial prefrontal cortex ( 724 , 757 ). Although it is not unlikely that such REM-related increases in dopamine activity modulate the effects of memory reprocessing during sleep (e.g., Ref. 73 ), the involvement of dopaminergic activity in sleep-associated memory consolidation has not been thoroughly examined thus far. Legault et al. ( 721 ) reported that the dopamine receptor blocker flupenthixol infused into the dorsal striatum 0–4 h after learning during “REM sleep windows” exerted the same impairing effect on habit consolidation as observed after scopolamine. In humans, so far only patients (with early Parkinson disease and schizophrenia) have been examined in this context with dopaminergic drugs that were partly rather unspecific ( 465 , 776 ). No consistent effects of the treatments on overnight retention of procedural or declarative memories were found in these patients.
Whether the very low levels of noradrenergic and serotonergic activity during REM sleep, perhaps in a permissive way, also contribute to memory consolidation, is currently not clear. Selective serotonin or norepinephrine reuptake inhibitors (SSRI or SNRI) that enhance availability of these monoamines in the synaptic cleft are commonly used for antidepressant therapy and, thereby, produce a substantial reduction in REM sleep. However, clinical observations revealed no clear memory impairments in patients treated with these drugs ( 22 ). Also, a more systematic clinical study of the effects of the SSRI citalopram and the SRNI reboxetine in moderately depressed patients failed to reveal any association of REM sleep diminution after the reuptake inhibitors, with decreases in overnight retention of declarative memories (word lists) or procedural skills (mirror tracing) ( 466 ). In healthy humans, administration of the SSRI fluvoxamine and the SNRI reboxetine during a postlearning period of REM-rich sleep likewise did not lead to any impairment in the sleep-dependent consolidation of mirror tracing or finger sequence tapping skills, although after the SNRI REM sleep was almost completely suppressed ( 962 ). On the contrary, sleep-dependent gains in finger sequence tapping accuracy were even significantly greater following the reuptake inhibitors than after placebo. The greater gains in accuracy after SNRI and SSRI administration were additionally correlated with increases in non-REM sleep spindle density. These findings challenge a role of phenotypic REM sleep for procedural memory consolidation. If any substantial contribution of REM sleep to procedural memory consolidation exists, increasing noradrenergic or serotonergic tone can apparently compensate for it. Both monoamines support synaptic remodeling via increasing activity of plasticity related immediate early genes ( 205 , 208 , 475 ).
It has been proposed that the inhibition of noradrenergic activity during REM sleep enhances procedural types of memory representations in cortico-striatal circuitry by enabling spontaneous reactivations in cortical networks that during wakefulness are under tonic inhibitory control by noradrenergic neurons ( 520 ). Similarly, it has been suggested that REM sleep allows for a replay of amygdala-dependent emotional memories that, in the absence of noradrenergic activation, decrease the emotional tone in these memories, whereas their information content is maintained ( 1294 ) (see sect. II C ). Additionally, the decreasing effect on emotional tone could be further enhanced by glucocorticoids, the levels of which are distinctly increased during late-night REM sleep and which distinctly diminish emotional memory consolidation ( 1272 ). However, there is presently little empirical support for these theories. Thus, overall, the role of noradrenergic and serotonergic inhibition during REM sleep for memory processing remains enigmatic.
VI. GENETIC APPROACHES TO SLEEP-DEPENDENT MEMORY FORMATION
Sleep is genetically controlled. Although environmental factors clearly contribute, a large part of the interindividual differences in sleep architecture is likely due to genetic factors. The search for genes involved in sleep regulation has received a great boost in recent years with the discovery of quiescent states in simple organisms like the fruit fly and worms that fulfill the criteria for sleep: a reversible and repeatedly occurring period of reduced responsiveness and relative inactivity which is homeostatically regulated (i.e., deprivation of sleep leads to subsequent longer sleep periods) ( 209 ). In these organisms, molecular methodology can be applied much more efficiently and at low costs mainly due to the reduced number of genes and neurons and the high reproduction rate. However, there are also important caveats, as certain sleep stages (e.g., REM sleep) and oscillatory brain activity characterizing sleep in mammals and humans cannot be discriminated in these simple organisms. Also, although typically homologs of genes exist between species, they can be much more differentiated in complex animals. For example, one Shaker gene in the fly has 16 homologs in rodents ( 202 ). These limitations underscore the importance of genetic studies in rats and mice and optimally in humans.
Gene expression studies, mainly in flies and mice, show an upregulation of genes specifically during sleep, including genes involved in protein synthesis and synaptic depotentiation. In addition, several genes related to neural plasticity and synaptic potentiation are upregulated during sleep depending on prior learning and exposure to novel experiences. Also, genes have been identified that exhibit joint regulatory actions on sleep and memory. Intriguingly, short-sleeping genotypes often also show impairments in learning and memory.
1. Differential gene expression during sleep and wakefulness
Early studies suggesting that gene expression differs between sleep and wakefulness mainly examined overall changes in mRNA and protein synthesis. First experiments showed that an injected radioactive substance was incorporated into newly synthesized RNA at a faster rate during sleep than during wakefulness ( 462 , 1257 ). Similarly, labeled proteins in rats increased after 90 min of sleep compared with 90 min of sleep deprivation ( 103 ). In rats and monkeys, the rate of protein synthesis correlated positively with the amount of non-REM sleep ( 848 , 956 ). Analysis of several hundreds of proteins by mass spectrometry ( 309 ) revealed that protein levels in the mouse cerebral cortex were generally decreased after sleep deprivation compared with sleep. These studies indicate that sleep favors protein synthesis, thus pointing towards a differential expression of genes during sleep and wakefulness. Indeed, blocking protein synthesis during sleep impairs sleep-dependent consolidation of ocular dominance plasticity (ODP) in the visual cortex of cats ( 1060 ) (see sect. VII A3 ), indicating that the increased protein synthesis during sleep has functional consequences for plastic processes underlying memory formation.
More recent studies have directly examined gene expression with molecular techniques like microarrays that indicate changes in transcription of the genome, i.e., the up- or downregulation for a great number of gene transcripts (for reviews, see Refs. 201 , 752 ). Cirelli and colleagues ( 204 ) examined gene expression in the cerebellar cortex of rats which were killed after 8-h periods of sleep, wakefulness, or sleep deprivation. Five percent of the examined transcripts were differentially regulated between sleep versus wakefulness or sleep deprivation. Sleep was associated with a great number of upregulated gene transcripts, and this number was indeed comparable with that during wakefulness, despite the behaviorally “inactive” state of sleep.
Importantly, one category of gene transcripts upregulated during sleep is involved in synaptic plasticity. This includes mRNA expression levels of calmodulin-dependent protein kinase IV (CAMK4), a gene that is implicated in synaptic depression and long-term memory consolidation ( 617 ), and the expression of several other genes associated with depotentiation and depression of synaptic strength. In contrast, during wakefulness, expression of genes that are involved in LTP is upregulated, including genes coding for Arc, c-Fos, NGFI-A, and BDNF ( 206 , 939 , 940 ). Based essentially on these gene expression patterns, the synaptic homeostasis hypothesis has been proposed assuming that wakefulness is associated with prevailing synaptic potentiation in cortical networks, whereas processes of synaptic depotentiation and depression predominate during sleep to desaturate the network ( 1204 ) (see sect. IV B ). Subsequent microarray studies in the mouse and fruit fly largely confirmed that expression of several genes is differentially regulated during sleep and waking and that these genes are related to synaptic plasticity, response to cellular stress, energy and lipid metabolism, and membrane trafficking ( 201 , 610 , 752 , 753 , 771 , 1357 ). In particular, the expression of genes involved in macromolecular synthesis increased during sleep, supporting a role for sleep in the synthesis of proteins and lipids (e.g., fats, sterols, vitamins) ( 753 ). Conversely, sleep deprivation strongly attenuates the expression of genes related to protein synthesis and thus downregulated translation in the mouse hippocampus, mainly mediated by key regulators of protein synthesis like mammalian target of rapamycin (mTOR) ( 1247 ). In the developing visual cortex in cats, expression of LTP-promoting genes (e.g., Arc, BDNF) was likewise found to decrease across sleep in the visual cortex as in adult rodents ( 1060 ) (see sect. VII A3 ). Notably, however, translation of the corresponding proteins at the same time was increased particularly during early sleep, suggesting that the first hours of sleep might be a period of accelerated protein synthesis in these networks.
Gene expression studies identified Homer1a as a core gene of sleep loss, as its expression was consistently upregulated in different strains of mice after 6 h of sleep deprivation ( 771 ). Homer1a expressing cells also overexpressed three further genes in response to sleep loss, which all appear to be implicated in intracellular calcium homeostasis, and may function to protect and recover neurons from glutamate-induced hyperactivity during periods of extended wakefulness. Even after controlling for possible confounding influences of increased glucocorticoids due to the sleep deprivation procedure, Homer1a (as well as plasticity-related genes including Arc and Fos) was still specifically associated with sleep loss ( 833 ). In the later study, several other genetic pathways that were previously associated with sleep homeostasis (e.g., circadian clock genes, see Ref. 415 ) were no longer affected by sleep deprivation in this study, indicating that effects of stress and glucocorticoids need to be carefully controlled in gene expression studies examining sleep homeostasis.
2. Experience-dependent local regulation of genes
Apart from the global regulation of gene expression during sleep and wakefulness, experience-dependent upregulation of plasticity-related genes has also been observed during sleep. Using fluorescence in situ hybridization in hippocampal cells in rats, Marrone and co-workers ( 781 ) found that exploration of a new environment increased the number of hippocampal CA1 neurons showing induction of the immediate early genes Arc and Homer1a both during exploration as well as during rest after exploration. However, others failed to reveal increased protein levels of c-Fos or Arc in cortical motor areas in rats during sleep following exploration or following training of skilled reaching, a task known to induce LTP in motor areas ( 510 , 584 ). Still, expression of the immediate early gene coding for BDNF increased following exploratory behavior during wakefulness, and the amount of exploration during waking (% recording time) was correlated with subsequent increases in BDNF measured in the frontal and parietal cortex after a short 10-min period of non-REM sleep, as well as with the amount of SWA during this non-REM sleep period ( 584 ).
Distinct local upregulation of plasticity-related genes and proteins following novelty and learning experiences has been revealed to be specifically related to REM sleep ( 261 , 1022 , 1225 ). Learning of a two-way active avoidance task in rats increased phosphorylation of the cAMP response element-binding protein (CREB), expression of Arc protein as well as mRNA expression of Arc, BDNF and early growth response-1 (Egr-1, also known as Zif268) in the dorsal hippocampus and amygdala during a 3-h postlearning sleep period. Elimination of pontine brain stem cells that generate P-waves hallmarking REM sleep suppressed retention of the avoidance response as well as learning-dependent increases in the expression of phosphorylated CREB, Arc protein, and mRNA of Arc, BDNF, and Egr-1 in dorsal hippocampus and amygdala ( 261 ). Conversely, cholinergic stimulation of P-waves by microinjection of carbachol into respective pontine regions increased expression of these proteins and mRNAs in the dorsal hippocampus, and was also associated with improved retention of the active avoidance response ( 263 , 794 ). These findings indicate that consolidation of an active avoidance response during REM sleep critically relies on P-wave-induced upregulation of synaptic plasticity-related genes in limbic regions, specifically in the dorsal hippocampus.
Exposure to novel tactile stimuli as well as experimental induction of hippocampal LTP during wakefulness produced an upregulation of Arc and Egr-1 in neocortical and hippocampal regions during subsequent REM sleep ( 986 , 987 , 989 ). The reinduction of both immediate early genes during REM sleep after novelty exposure was only transient in hippocampal regions, and more pronounced and persistent in the neocortex, where it was particularly strong in the somatosensory areas most activated by the previous tactile novelty experience ( 986 , 989 ). Cortical expression of Arc was correlated to EEG spindle activity during prior non-REM sleep, possibly favoring immediate early gene activity during REM via Ca 2+ -dependent mechanisms ( 989 ). Spindles likely promote massive calcium influx into cortical pyramidal cells and through activation of CaMKII may set the stage for the expression of immediate early genes during ensuing REM sleep ( 283 , 1061 ). Both Arc as well as Egr-1 are known to interact with CaMKII in synaptic remodeling during LTP. Ribeiro et al. ( 989 ) related the REM sleep-associated increase in immediate early gene expression occurring preferentially in cortical over hippocampal regions to waves of synaptic plasticity that hippocampus-dependent memories undergo during successive non-REM-REM sleep cycles (see FIGURE 7 , A AND B ). Reactivation of hippocampal memory representations during non-REM sleep stimulate the redistribution of these memories to neocortical areas where they are synaptically consolidated during ensuing REM sleep ( 293 ). Across several waves of plasticity associated with the non-REM-REM sleep cycles, memories become stored mainly within neocortical networks while they fade out in the hippocampus, the latter coinciding with the fading of reinduction of immediate early gene activity during postencoding REM sleep in hippocampal areas ( FIGURE 7 C ) .

Different time courses of plasticity in the hippocampus and neocortex. A : concept: the hippocampus undergoes a few plasticity waves before fading out. These plasticity waves are probably enough for memories to remain in the hippocampus for weeks or months. In contrast, the cerebral cortex undergoes plasticity waves for a much longer period of time, leading to many more cycles of memory reinforcement and years-old memories. B : in single neuron recordings in rats, long-lasting firing rate increases after novel spatio-tactile stimulation (EXP) occurred during SWS in primary somatosensory cortex (S1), but not in the hippocampus (HP) or primary visual cortex (V1). Increased neuronal activity persisted for hours after experience offset during SWS in S1. Shown are the normalized firing rates during concatenated SWS episodes spanning an entire representative experiment. Ticks at the bottom indicate SWS episode boundaries. C : model of memory propagation from hippocampus to neocortex during sleep. Via thalamo-cortical inputs (not shown), episodic and spatial memories are acquired during waking as new synaptic changes (red) distributed over hippocampocortical networks of neurons ( top panel ). The recurrence of cortical plasticity during subsequent sleep causes the stabilization and propagation of new synaptic changes in the neocortex. Conversely, the fast decay of sleep-dependent plasticity in the hippocampus generates a net outflow of information, gradually flushing memories to associated cortical networks over time. [Modified from Ribeiro et al. ( 989 ).]
3. Deletion and gene knockout studies
In flies, several genes that regulate the amount of sleep have been identified by mutagenesis screenings. Whereas wild-type flies sleep ∼8–10 h/day, flies with a loss-of-function mutation in the genes Shaker (Sh) or hyperkinetic (Hk) sleep only 2–4 h/day ( 154 , 202 , 203 ). Shaker codes for the alpha subunit of a specific K + channel (alpha-subunit of a tetrameric potassium channel that mediates a voltage-activated fast inactivating IA current), and hyperkinetic codes for a regulatory beta-subunit of this channel that interacts with the alpha-subunits encoded by Shaker ( 202 , 1052 ). Shaker-modulated K + channels have homologs in vertebrates (Kv.1 and other Shaker-like channels, Kv.2, Kv.3, Kv.4, etc.). In both flies and mammals, they play a major role in membrane repolarization and likely also modulate EEG oscillatory phenomena like spindles ( 35 , 155 , 244 , 320 , 357 , 358 , 717 , 934 , 1266 ). Importantly, both Shaker and hyperkinetic mutants showed marked impairments in learning and memory, as well as a reduced life span. Similarly, reduced sleep and life span have been reported for flies with a loss of the gene Sleepless (SSS) which codes for glycosylphosphatidylinositol-anchored protein with unknown function, with the effects of this mutation possibly in part also mediated by Shaker channels ( 202 , 272 , 653 , 1306 , 1337 ). However, its influence on learning and memory has not been investigated so far.
There is strong evidence for an involvement of clock genes in sleep-dependent plasticity. Clock genes regulate the circadian rhythm and affect sleep ( 681 ). In mammals, genetic variations in both clock genes activating circadian rhythm, like Clock (Clk) and cycle (cyc), and clock genes with repressing functions on circadian rhythm, like period (per) and Cryptochrome (Cry), have been linked to variations in sleep ( 663 , 703 , 851 , 1073 , 1328 , 1329 ), whereas in flies only the activators Clk and cyc affect sleep phenotypes ( 531 ). Per, which is regulated by CREB, plays a key role in long-term memory formation in Drosophila ( 1023 ). Recent studies in fruit flies by Shaw and colleagues revealed that per, together with other clock genes, also regulates sleep-dependent memory formation ( 314 , 438 ). These studies first established that waking experience, i.e., a socially enriched environment where the flies were housed in groups of ∼40, compared with social isolation increased sleep time and sleep bout duration ( 315 ). Sleep need likewise increased after a learning task (conditioned suppression of courtship behavior), and this increase in sleep was critical for successful recall of the conditioned behavior 48 h later ( 314 , 438 ). Remarkably, flies mutant in the clock gene per failed to show this experience-dependent increase in sleep need and in parallel showed no long-term memory after 48 h. Similar results were observed in flies with mutations in the rutabaga gene (involved in cAMP signaling) and blistered (involved in synaptic LTP and contextual habituation). Rescue of the genes in clock neurons of the flies reestablished the experience-dependent increase in sleep as well as long-term memory ( 314 ).
Several other genes and transcripts have been identified that not only strongly influence sleep quantity, but also are involved in major pathways regulating synaptic plasticity, like CREB ( 477 , 532 , 916 ), the extracellular signal-regulated kinase/mitogen-activated protein kinase (ERK/MAPK), and the epidermal growth factor (EGF) (e.g., Ref. 401 , for a review see Ref. 202 ). However, direct links between sleep and memory formation have not been demonstrated for most of these signals. An exception is the gene bunched, a regulator of the transmembrane receptor Notch which is involved in both sleep homeostasis and learning in flies ( 1062 ). Overexpression of the Notch ligand Delta as well as the introduction of a Notch gain-of-function allele reduced sleep rebound and prevented impairments in new learning (aversive phototaxic suppression) induced by prior sleep deprivation. Interestingly, the effects on sleep and memory of Notch signaling were mainly observed in glia cells, pointing to a critical role of neuron-glia interactions for regulating sleep-dependent learning benefits ( 1338 ). Similar effects of Notch signaling were observed in worms ( Caenorhabditis elegans ) ( 1085 ).
Knockout procedures have also been used in numerous studies as a genetic tool to examine the impact of neurotransmitter systems on sleep regulation (reviewed in Ref. 202 ; see also sect. V B ) and oscillatory EEG activity during sleep (reviewed in Ref. 37 ; see also sect. IV). For example, a genetic region on chromosome 13 (containing several genes) was identified by quantitative trait loci analysis (QTL) that explained almost 50% of the variance in the rebound in non-REM sleep EEG delta activity after sleep deprivation in rodents ( 414 ). However, for none of these genes have any links to sleep-dependent memory formation been examined in a more systematic manner.
3. Heat-induced sleep in transgenic animals
Gerstner and co-workers ( 450 – 452 ) engineered heat-responsive transgenic flies to examine the role of fatty-acid binding proteins (Fabps) for sleep-associated memory consolidation. Fabps bind small lipids and act as transporters in various cells and tissues. Of the nine mammalian genes, mRNA coding for Fabp7 is expressed in the brain, and in rodents its expression follows a circadian rhythm. In flies, mRNA expression coding for the Fabp7 homolog dFabp also shows a circadian rhythm, with elevated levels during the night. Inducing mRNA expression of genes coding for Fabp7 or dFabp in temperature-sensitive transgenic flies by elevating ambient temperature from 20 to 30°C increased sleep and, if temperature was raised after learning, had an enhancing effect on long-term memory for a conditioned olfactory avoidance response. While baseline sleep was lowered in the transgenic flies, the heat-induced increase in sleep was correlated with the gain in memory, altogether suggesting an important role for Fabps in mediating memory consolidation during sleep ( 451 ).
Using a similar approach, Donlea et al. ( 316 ) introduced a temperature-sensitive cation channel in neurons of Drosophila . Activating the channel by raising temperature above 31°C resulted in a sleeplike state. Inducing 4 h of sleep in this way after massed trials on a courtship conditioning procedure enhanced retention of the conditioned behavior at a retest 48 h later, compared with flies that did not sleep after training. The effect cannot be explained by an activation of the temperature-gated neurons per se, because sleep deprivation during periods of high temperature did not enhance long-term memory. Of note, temperature-induced sleep also benefited new learning on the next day, fitting the idea that memory consolidation during sleep is concurrently linked to processes preparing the brain for the future encoding of new information ( 828 ).
These studies exemplify the rapid development of new genetic tools that can be used as the “remote control” of sleep ( 316 ) and, in this way, to study the function of sleep for memory consolidation. First attempts conducted to control sleep optogenetically in mice have revealed that fragmenting postlearning sleep by activating hypothalamic hypocretin/orexin neurons impaired consolidation in a novel object recognition task ( 9 , 1009 ). Future developments of these transgenic techniques will enable the reliable activation of specific neuronal memory traces, thus providing promising tools to a more fine-grained analysis of the fate of a memory trace during sleep ( 739 ).
1. Stability and heritability of sleep
Human sleep and particularly oscillatory EEG activity during sleep is highly heritable and very stable within individuals ( 689 ). In addition, large interindividual differences in the sleep EEG exist, which are more than 10 times higher than those, observed within subjects across multiple nights of sleep. Already in 1937, Geyer ( 453 ) reported a higher similarity in sleep profiles for monozygotic compared with dizygotic twins. Systematic twin studies using large samples indicate that genetic differences account for 30–45% of the variance in subjective sleep quality and sleep disturbances ( 186 , 526 , 889 ) and for ∼50% in physiological measures of sleep stages ( 137 , 737 , 738 ). Highest heritability values are observed for REM density (h 2 = 64–95%) as well as EEG spectral power particularly in the alpha (8–11 Hz) and spindle (11–15 Hz) frequency bands (h 2 = 76–96%) ( 29 , 266 , 444 , 736 , 1227 ). Similar heritability estimates (h 2 = 40–60%) have been reported for sleep amount and sleep organization in rodents ( 1165 , 1166 , 1226 ), with even higher estimates of genetic control for oscillatory electrical activity during sleep ( 37 , 414 , 416 , 417 ).
Genetic factors also contribute to sleep differences between ethnicities (e.g., Ref. 1019 , for a meta-analysis) and gender (e.g., Ref. 976 ). Women sleep longer and still have a higher SWS percentage than men, whereas men show increased non-REM sleep stages 1 and 2 ( 424 , 587 , 652 , 952 ). In a study in 2,600 participants aged 37 to 92, gender explained 14.6% of the variance in SWS percentage and 10.9% of stage 2 sleep percentage ( 976 ).
Additionally to the strong heritability, the human sleep EEG is remarkably stable within individuals across multiple nights ( 151 , 1176 , 1177 , 1201 ). Stability as indicated by intraclass coefficients is highest for SWS (73%), but also significant for other parameters like the amount of REM sleep (48%), stage 2 sleep (56%), and total sleep duration (46%). Power in the delta frequency band (0.75–4.5 Hz) shows particularly high stability (78–89%). Interindividual differences in delta power were on average ∼10 times greater than the rebound in delta activity observed following sleep deprivation ( 818 , 1213 ). The interindividual differences in the sleep EEG are remarkably robust also against sleep disturbances, first night effects, prior sleep deprivation, and the administration of sleep-promoting agents ( 881 , 1213 ).
2. Genetics, sleep and cognitive function
Specific genetic markers of sleep have been identified mainly in studies of different populations with disordered sleep, such as fatal familial insomnia, narcolepsy, restless legs syndrome, and circadian rhythm disorders ( 266 , 299 , 638 , 954 , 1058 , 1167 ). However, the relationships of these genetic markers to sleep-dependent memory processes are entirely unknown so far, except one observation indicating that a single nucleotide polymorphism (SNP) in the prion protein gene (PRNP) implicated in fatal familial insomnia in human and sleep regulation in mice ( 579 ) affects learning performance in healthy participants ( 886 ).
Studies in healthy humans revealed clear associations between sleep and genes involved in regulating circadian rhythm, mainly the clock gene PER3 that can occur in a different number of repetitions, so-called “variable number of tandem repeats” (VNTR) polymorphisms. Homozygosis for the 5-repeat allele (PER3 5/5 ) has been repeatedly associated with morning preferences (morning types) compared with homozygotes for the 4-repeat allele (PER3 4/4 ) and heterozygotes (PER3 4/5 ) ( 41 , 61 , 349 , 609 , 874 , 917 ), as well as with increased SWS percentage and SWA during non-REM sleep and increased theta power during REM sleep and wakefulness ( 1256 ). In addition, PER3 5/5 carriers were more vulnerable to sleep deprivation in the early morning hours (e.g., Refs. 1256 and 483, but see Ref. 467 ) and exhibited a stronger decrease in brain activity assessed by fMRI in prefrontal areas from morning to evening ( 1244 ). Altogether, these findings suggest that circadian genotypes contribute to differences in the allocation of cognitive resources, thus possibly also affecting memory processing during sleep.
With regard to noncircadian genes, Landolt and co-workers identified a genetic difference (G->A transition at codon 22) in the gene encoding the adenosine metabolizing enzyme (adenosine deaminase, ADA) that was significantly associated with SWS duration and SWA, in particular in the <2 Hz range (e.g., Refs. 54 , 798 , 982 ; but see Ref. 797 ). A-allele carriers who exhibited more SWS also showed poorer performance in focused attention (as indicated by the d2 test), but not in several other tests of short-term memory and executive functions (verbal, figural memory, digit span, Stroop test. etc.) (see Ref. 688 for a review).
Differences in the widely researched functional Val158Met polymorphism in the gene coding for the catechol- O -methlytransferase (COMT) were associated with more global alterations in alpha peak frequency as well as in spectral power in the 11–13 Hz band, occurring during REM, non-REM sleep, and also during wakefulness ( 104 , 105 ). Investigation of the Val66Met polymorphism, i.e., a valine to methionine amino acid substitution at codon 66 of the BDNF gene, revealed that Met-allele carriers (compared with Val/Val homozygotes) exhibit less SWS and reduced delta and theta activity during non-REM sleep in baseline nights as well as in response to sleep deprivation ( 54 ). BDNF is particularly expressed in the prefrontal cortex and the hippocampus in humans ( 927 ), and the Val66Met BDNF polymorphism has been repeatedly associated with a range of cognitive functions including memory (e.g., see Ref. 614 , for a meta-analysis). Unfortunately, none of these studies included direct tests of sleep-dependent memory formation.
3. Genetic studies on sleep-dependent memory consolidation
Studies mainly on flies indicated that similar genes are involved in sleep regulation and memory processing, and that memory and plasticity-related genes are expressed differentially during sleep and wakefulness. No such studies are available in humans. In addition, no genome-wide association study in humans with regard to sleep and memory parameters has been conducted thus far, although such studies would be highly informative. In fact, presently, direct evidence for a genetic contribution to the sleep-dependent formation of memory is entirely lacking.
There is a strong genetic influence on sleep parameters (discussed above) and also on memory formation. Twin studies have estimated that 50% of the variance in learning performance, typically measured after short retention periods of 5–30 min, is due to genetic factors ( 127 , 885 , 1164 , 1262 ). Against this background, as both sleep and memory measures strongly differ among individuals, a putative genetic determination of sleep-dependent memory consolidation should basically express itself in significant correlations between the respective sleep and memory parameters on the interindividual level. Hence, the demonstration that sleep and memory processes share significant portions of their interindividual variance represents a first and most important step towards the demonstration of any genetic contribution to sleep-associated memory formation, although it does not prove such contribution because the associations can basically result from parallel, but independent, influences of the putative genetic factor on the sleep and memory processes of interest. Also, it cannot be concluded from interindividual sleep-memory associations whether a putative genetic factor primarily affects sleep to change memory processing or, conversely, affects memory processing to change sleep, although clues can be derived from temporal relationships. For example, Dionne et al. ( 310 ) showed in a longitudinal twin study that longer and more consolidated sleep at the age of 6 mo predicted better language development at 18 and 30 mo. As the type of sleep was highly heritable, the findings suggest a sleep-mediated genetic influence on later language proficiency.
With regard to non-REM sleep, spindles have been consistently revealed to be associated with memory-related parameters and thus represent a most promising candidate mediating a genetic link between sleep and memory. In children and adults, spindle number and density strongly correlate with IQ scores ( 106 , 395 , 439 , 847 , 1038 , 1039 ) as well as overnight retention of memories ( 213 , 1057 , 1230 ). Abnormally large spindles or the absence of spindles were observed in mentally retarded and dyslexic children ( 93 , 148 , 400 , 455 , 456 , 1070 , 1071 ). Contrasting with the findings in healthy humans, elevated baseline spindles predicted poor performance on a two-way shuttle box avoidance task in rats ( 397 ). However, learning-dependent increases in sleep spindles only occurred in rats that successfully learned the task, and these increases were positively correlated with post-sleep improvements in performance. Based on these findings, Fogel and Smith ( 398 ) proposed a curvilinear relationship between sleep spindles and capabilities of learning, with high spindle numbers reflecting either highly efficient or pathological memory processing in thalamocortical systems.
Although the importance of SWA and slow oscillation for memory processing is well established (see sect. IV A ), interindividual correlations between these EEG oscillations and learning capabilities and intelligence have been less frequently reported. This is remarkable given the great interindividual variance and intraindividual stability of these sleep EEG parameters (discussed above). Several studies reported correlations between SWS or SWA and the overnight retention of declarative memory ( 55 , 58 , 464 , 1319 ) as well as procedural motor skills ( 581 , 582 ). However, because in these studies the sleep EEG was assessed after the learning phase in highly homogenous subject samples, learning-induced changes in EEG activity could not be clearly dissociated from trait-dependent variance.
Historically, memory function has been much more often associated with parameters of REM sleep than non-REM sleep. Evolutionary increases in encephalization are positively associated with sleep time allocated to REM sleep, even when the effects of phylogenetic similarity between species are controlled (e.g., Ref. 725 , but see Refs. 1077 , 1078 ). Analyses of sleep in seven inbred strains of mice revealed a high correlation between the relative and absolute time in REM sleep and the performance level avoidance conditioning or maze learning across strains ( 880 ). In humans, mentally retarded children generally exhibit less REM sleep, longer REM sleep latencies, and less REM density relative to normal controls ( 182 – 184 , 211 , 368 , 369 , 425 , 922 – 924 , 1045 ). However, correlations between REM sleep and IQ measures in healthy children and adults revealed mixed results ( 126 , 153 , 922 , 923 , 1183 ). In some studies, gifted children tended to sleep longer and had more stage 2 sleep, compared with controls ( 153 , 1183 ). In addition to baseline amounts of REM sleep, the magnitude of learning-induced increases in REM sleep parameters have been linked to capabilities of learning in animals ( 1110 ) and humans ( 1114 ).
The studies discussed so far examined associations between sleep and memory in rather small samples (12–30 participants). Together with the testing of multiple sleep parameters (like amounts of SWS, REM sleep, spindle numbers, density, etc.) and with multiple measures of memory and learning capabilities, this can lead to an overestimation of the strength of associations due to multiple comparisons. To overcome such limitations, in recent study, we assessed sleep (at home by portable polysomnography) together with measures of pictorial and procedural motor learning and overnight memory retention in a large sample of 855 young healthy participants (Ackermann, Pappassotiropoulos, De Quervain, and Rasch, unpublished results). In accordance with previous studies, the number of fast spindles (13–15 Hz) showed a positive (but rather small) correlation with short-term recall (tested after 10 min) of pictures. However, this correlation was entirely explained by gender differences, as women remembered more pictures and exhibited also an increased number of spindles. Short-term recall of pictures was also correlated with the percentage of REM sleep ( r = 0.09), with this correlation surviving control for gender. Also, with regard to overnight retention of pictures, the only significant correlation was revealed for time in REM sleep: increased REM sleep was associated with diminished overnight retention of pictures ( r = −0.13), independently of whether these pictures were emotional or neutral. No such associations were observed for SWS-related measures, spindles, or sleep length. Overall, these data indicate a surprisingly weak association between interindividual differences in sleep and memory parameters. Specifically, they suggest that the genetic determination of sleep, particularly of SWS-related measures and spindles, does not substantially contribute to sleep-dependent memory formation processes, as these parameters do not appear to be associated with each other on the interindividual level. It is important to note, however, that the lack of association between sleep and memory parameters among subjects does not at all rule out a basic role of sleep in memory formation. For example, one individual can display higher SWA than others due to neuroanatomical and related genetic factors in the absence of any differences in memory among these individuals, yet increasing SWA in this same individual might still lead to a distinct improvement in memory consolidation during sleep.
VII. DEVELOPMENTAL ASPECTS OF SLEEP-RELATED BRAIN PLASTICITY
Only a handful of studies have been conducted to explore memory-consolidating functions of sleep during early postnatal periods, infancy, and adolescence in humans. The developmental approach might be particularly important for understanding the mechanisms underlying sleep-dependent memory consolidation, mainly for two reasons. First, compared with adults, infants and children sleep longer and deeper. In parallel, during early life the brain exhibits particularly strong plasticity shaping memory systems and underlying neuronal circuit formation. During limited periods early during development, the brain is particularly sensitive to certain experience that instructs neuronal circuits to represent respective information as very persistent memories determining performance throughout later life ( 650 ). However, the mechanisms underlying neuronal plasticity during such critical periods differ from that during adulthood. As a consequence of maturational processes, critical period plasticity is linked to a distinct biochemical milieu, a change in the subunit composition of NMDA receptors in forebrain synapses from a predominance of NR2B subunits at birth to a prevalence of synaptic NR2A subunits towards the end of the critical period, a specific adjustment of the balance between excitatory and inhibitory inputs to neuronal networks, and eventually also to different features of spike timing-dependent plasticity ( 332 , 352 , 545 , 838 , 1212 ). The specific characteristics of plasticity during critical periods prevent a straightforward generalization of the effects of sleep on plasticity in the adult brain. Nevertheless, it is striking that studies of the developing brain, including those targeting critical period plasticity, show an influence of sleep on the formation of memory that is even more profound than that observed in the adult brain.
A. Early Development: Animal Models
Filial imprinting in domestic chicks, song learning in zebra finches, and ocular dominance plasticity in the visual cortex of cats have been studied as models of developmental brain plasticity and memory formation in animals in conjunction with sleep. Recent research has indicated that the formation of these three types of memory indeed critically depends on sleep.
1. Filial imprinting
Imprinting is a very strong and early form of social recognition memory, often studied in the domestic chick. Under natural conditions, during imprinting the chick learns the characteristics of its mother to selectively follow her rather than any other adult. In the laboratory, the chick is repeatedly exposed to a moving object, and so acquires a preference for this object over an alternative, novel stimulus, with this preference used as measure of the imprinting memory ( 107 , 567 ). Imprinting over repeated training sessions leads to a gradual increase in the number of neurons in the intermediate and medial mesopallium (IMM, also termed intermediate and medial hyperstriatum) that selectively respond to the imprinting stimulus which is correlated with the chick's preference for the imprinting stimulus ( 144 , 568 ). Imprinting increases also the amount of activated CaMKII, the size of dendritic spine postsynaptic densities, and a delayed upregulation of NMDA receptors in the IMM, without changing the number of synapses per se ( 129 , 567 , 799 ).
An involvement of sleep in imprinting was suggested by an early study ( 1120 ) in which a single imprinting session starting 12 h after ecclosion produced a significant increase in the number of episodes and the amount of REM sleep, whereas after pseudo-imprinting REM sleep decreased. In a different study, changing the imprinting object from one day to the other produced a bias towards increased left hemispheric sleep, possibly related to increased consolidation processes in the left hemisphere ( 102 ). Unilateral sleep typically covers ∼1–2% of sleep time in chicks. Results from a more recent study compellingly demonstrated a critical involvement of sleep in the consolidation of the imprinting stimulus ( 600 ). In this study, one group of chicks was allowed to sleep undisturbed after imprinting training for 6 h before being retested and then was subjected to a 6-h period of (slightly) disturbed sleep. Final testing after the second sleep period revealed a stable memory for the imprinting stimulus together with an overall doubled number of IMM neurons responding to the imprinting stimulus (compared to imprinting training before sleep). In contrast, chicks that experienced disturbed sleep in the first 6-h interval after training and undisturbed sleep in the second 6-h interval, at final testing did not exhibit any significant memory for the imprinting stimulus and showed a strong decrease in the number of IMM neurons responsive to the imprinting stimulus. In addition, chicks with undisturbed sleep after imprinting displayed an increase in EEG SWA covering the 0–6 Hz frequency band during this period. These findings point to a particular importance of SWS, occurring in a window ∼1.3–5 h after stimulus exposition, for forming a stable memory for the imprinting stimulus. Interestingly, tracking of individual IMM neurons revealed a pattern suggesting that sleep shortly after imprinting training stabilizes the memory representation by supporting the recovery of responsiveness of cells that for any reasons had ceased to respond to the imprinting stimulus in the course of training or shortly afterwards, with this effect requiring some hours to occur ( 600 , 1150 ). The mechanisms of this sleep effect are unclear. Given that the IMM corresponds to parts of the mammalian neocortex receiving significant input from the hippocampus ( 819 , 979 ), such inputs during sleep may drive the stabilization of IMM representations, although hippocampal neurons do not appear to selectively respond to the imprinting stimulus after training ( 600 , 858 ).
2. Song learning in birds
Song learning in birds was one of the first models used to systematically study the role of sleep in developmental learning. It bears great similarity with speech learning in human infants ( 321 , 774 ). The surprising discovery that sleep is essential to a bird's acquisition of a song fostered a major advance in this field ( 773 , 774 ). Song learning in birds has been mainly studied in zebra finch males which develop their song between day 30 (after hatching), when they start producing unstructured sounds, and day 90, when they exhibit a well-developed song to be used as a complex social signal ( 321 ). Song learning is based on an innate predisposition to imitate vocalizations, and requires exposure to a song model. Under experimental conditions, the birds are typically subjected to a standard protocol, i.e., they are first raised by females who do not sing and then, at the appropriate age, gain limited access to a tutor song by a form of instrumental conditioning, e.g., by pecking a certain key ( 774 , 1181 ). Song learning has been conceptualized as a two-stage process ( 660 , 661 ): first, the bird acquires a sensory model (“template”) of the tutored song which in a second sensorimotor step the bird gradually learns to imitate, whereby auditory feedback is essential for this process.
Derégnaucourt et al. ( 279 ) provided compelling evidence that the sensorimotor phase of song development is driven by sleep. Monitoring the structure of the song and its syllable features by frequency-based automated song analyses across the whole period of song development in juvenile zebra finches, they revealed that song structure profoundly deteriorated after nocturnal sleep compared with the evening before sleep, and was regained only after intense morning singing ( FIGURE 8 A ) . The effects occurred on the background of a gradual day-to-day increase in song structure and were not seen in adult birds. Interestingly, the young birds showing the greatest morning deterioration in song structure achieved the best final imitation of the tutored song at the end of the 45-day monitoring period, suggesting a functional role of the sleep-related deterioration in singing behavior for the overall learning process. Deterioration of song structure occurred also after induction of sleep during the daytime by the administration of melatonin excluding confounding effects by the circadian rhythm.

Sleep-dependent formation and reactivation of song memory in birds. A : the measure of Wiener entropy variance (EV) of song structures reveals a continuous improvement in song structure in young birds over the 45-day developmental period starting with the first exposure to the tutor song (start of training). In spite of this overall improvement, during early development (day 46, middle panel ) overnight sleep induces an acute decrease in song performance as indicated by a stronger deviation in song structure in the morning after sleep compared with presleep performance. This overnight decrease is not any more present in the end of the learning period (day 89, right panel ). Bottom panel indicates continuous tracking of EV values over the 45-day period. [Modified from Derégnaucourt et al. ( 280 ), with permission from Nature Publishing Group.] B : neuronal trace of an arcopallium (RA) neuron emitting 10 distinct bursts of 2–7 spikes/burst (”singing“). The bursts are precisely timed to when the bird sang a song whose motif consisted of a sequence of five syllables (see spectrograph, frequency vs. time representation; top ). For each song bout, the sequence of syllables and the structure of each spike burst (timing of spikes and numbers of spikes) were highly reliable. The same pattern of spike-bursts reoccurred during recording during sleep. [Modified from Dave and Margoliash ( 267 ), with permission from American Association for the Advancement of Science.]
The neuronal mechanisms during sleep that mediate the deterioration in morning song structure are not clear. The major auditory and song system pathways of the songbird brain have been described in terms of a functional hierarchy. The caudal medial nidopallium (NCM) and the caudal mesopallium (CM) receiving inputs from primary auditory structures contribute to the formation of auditory representations likely including those forming the sensory template of the tutored song ( 108 , 463 ). These structures represent a major source of the inputs to the vocal control “song system” comprising chiefly the nucleus HVC (“high vocal control”) and the robust nucleus of the arcopallium (RA) representing the motor cortex analog of the song system ( 774 ). Along with the deterioration of song structure, HVC neurons show a decline in burst activation across sleep in juvenile songbirds.
In adult songbirds, RA premotor neurons have been identified that show replay during sleep, i.e., patterns of burst activity during sleep which, in terms of their temporal sequence and spike sequence structure within each burst, are very similar to those observed during singing ( FIGURE 8 B ) . The replay in RA neurons is driven by input from HVC neurons ( 267 , 504 ) which, in juvenile songbirds, show a decline in burst activation across sleep, in parallel with the deterioration of song structure ( 271 ). Several studies by Margoliash's group provided evidence that the replay activity during sleep indeed reflects a processing of memory representations producing changes in the representation and also in subsequent song performance. Thus, in juvenile zebra finches, the first exposure to a tutor song distinctly enhanced high-frequency bursting in RA neurons during subsequent sleep, with corresponding changes in singing occurring not until the following day ( 1063 ). Moreover, the sequence structure of spiking within bursts identified during sleep was significantly correlated with that observed while the bird was listening to the tutor song during the preceding wake phase. Both the increase in burst occurrence and burst structure in RA after initial exposure to the tutor song also required that the bird receive auditory feedback from its own singing, as these increases were drastically reduced when the birds were experimentally deprived from auditory feedback or were surgically muted.
The findings suggested a model where RA burst activity during sleep and the forming of sensorimotor representations of a song requires a twofold of inputs during waking, i.e., on the one hand, auditory feedback activity from actual singing during waking that serves a permissive role in structuring night-time RA bursting and organizing the song representation and on the other hand activity related to the sensory template of the tutor song ( 774 , 775 ). Given that RA burst activity during sleep reflects sensory experience of the tutored song during the wake phase, reactivated “template” activity during sleep may drive plastic changes in the song representation that, as they occur under unsupervised conditions (i.e., in the absence of actual auditory feedback), may manifest themselves in a deterioration of song structure in the morning after sleep ( 280 , 463 , 856 , 857 ). Early during song development, reactivated sensory representations and sensorimotor feedback as experienced during actual singing are poorly correlated, resulting in large differences in singing between morning and evening performance which disappear with increased learning. In addition to modifying sensorimotor representations, reactivations during sleep may also strengthen the song template ( 136 ). Whether SWS or REM sleep is more important for the processing of song memories during sleep cannot be answered, as RA burst activity appeared to be equally affected in both sleep stages ( 1063 ). In addition, it remains unclear whether sleep actually benefits reorganization of memory traces between brain structures as assumed for episodic memories in humans ( 964 , 965 ). Although this model as proposed by Margoliash and co-workers ( 774 , 775 ) aims to explain developmental song learning, it may account also for song learning and the maintenance of learned songs in adult songbirds. There is evidence for song replay and for systematic changes in RA burst activity patterns across sleep in adult zebra finches as well ( 969 ). Interestingly, RA burst activity during sleep in some of these cases appeared to be more similar to that observed during singing after sleep than before sleep, suggesting a kind of “preplay” that occurs during sleep and creates new song features ( 323 ).
3. Ocular dominance plasticity
Ocular diminance plasticity (ODP) has been studied mainly in cats as a developmental model of synaptic plasticity that is induced by specific stimulus conditions, i.e., monocular deprivation ( 77 , 411 ). In critical periods during development (in cats between about postnatal days 28 and 40), blocking vision in one eye causes a massive rewiring of cortical circuitry in favor of the open eye. ODP is sleep-dependent inasmuch as sleep following a period of monocular deprivation resulted in an almost twofold increase in synaptic remodeling in visual cortical areas, whereas after a similar waking interval in complete darkness, a tendency for erasure of the effects induced by the preceding monocular deprivation was observed ( 412 ). The beneficial effect of sleep on consolidation of ODP mainly leads to a strengthening of the cortical responses to stimulation of the nondeprived eye ( 48 ). Inactivating the sleeping visual cortex by administration of the Na + channel blocker lidocaine or the GABA A agonist muscimol inhibited ODP, indicating that postsynaptic activity during sleep is required for consolidating the plastic changes induced by a monocular experience ( 411 , 605 ). Sleep-dependent ODP is prevented by blocking glutamatergic NMDA receptors or cAMP-dependent protein kinase (PKA) during sleep after monocular deprivation ( 48 ). Blockade of NMDA and PKA signaling was associated with reduced activation of the kinases CaMKII and ERK as well as phosphorylation of GluR1 at Ser831, i.e., processes that are critical to the insertion of AMPA receptors into the postsynaptic membrane and the strengthening and maintenance of LTP. Most recently, Seibt et al. ( 1060 ) demonstrated the critical dependence of ODP on protein synthesis during sleep, as respective plastic changes in visual cortex could be prevented by inhibition of mTOR-mediated protein synthesis through rapamycin. Rapamycin had no effect on plasticity induced during wakefulness. Furthermore, phosphorylation of regulators of protein synthesis as well as translation of plasticity-related mRNAs (e.g., Arc and BDNF) was increased during sleep, overall speaking for the notion that sleep is a period of enhanced protein synthesis in these networks. ODP was paralleled by increased multiunit activity during both SWS and REM sleep, and was correlated to the time spent in non-REM sleep ( 412 ). Of the GABA A agonistic substances zaleplon, eszopiclone, zolpidem, and triazolam, only zolpidem impaired sleep-dependent ODP, although all substances distinctly reduced REM sleep and increased non-REM sleep ( 48 , 1059 ). The differential efficacy of these substances in diminishing ODP may be related to differences in their pharmacodynamics and selectivity for binding specific GABA A receptor subtypes, but is independent of their profound changes in sleep architecture, as determined by standard EEG sleep recordings. ODP was impaired following application of the atypical hypnotic trazodone, probably acting via blocking 5-HT 2c receptors during sleep ( 48 ). Since serotonin activity is at a minimum during REM sleep and at intermediate levels during non-REM sleep, this finding suggests that processes during non-REM are more important for the expression of ODP. Interestingly, there is evidence that ODP critically depends on the T-type calcium channel function ( 1224 ), which also plays an essential role in the generation of spindles and slow oscillations during non-REM sleep ( 282 , 283 ).
In sum, this research indicates that sleep plays an essential role for behaviors that are formed during critical or sensitive periods in early postnatal life. The mechanisms mediating synaptic plasticity during critical periods in essential aspects differ from those during later life ( 838 ) and differences in the regulation of synaptic plasticity may still exist between juveniles and adults ( 772 ). Yet, this does not necessarily imply that the manner in which sleep contributes to critical period plasticity also basically differs from the effect of sleep on memory formation in the adult brain. Sleep during the early postnatal period and in adults mainly differs in quantity of total sleep, time in SWS and REM sleep, intensity of SWS and SWA, etc., suggesting that the impact of sleep on memory formation during early development is overall stronger than during adulthood, rather than differing in quality. This view may account especially for developmental learning processes that are less clearly linked to an early postnatal critical period but extend towards adolescence, like song learning in birds or language learning in children. Indeed, the power of sleep in forming memory during early life and the fact that these memories are the first to be formed may suffice to explain the unique strength of these memories being difficult to overwrite or reverse.
B. Early Development: Human Studies
Investigations of the effects of sleep on memory formation during early development in humans focused on declarative and procedural memory processes similar to those examined in adults, rather than on behaviors linked to sensitive periods, like social imprinting and amblyopia as a consequence of monocular deprivation. Overall, these studies point towards the particular relevance of SWS for sleep-dependent memory consolidation in children. In adults, SWS has been revealed to play a causal role for the consolidation of hippocampus-dependent declarative memories ( 783 , 957 ), but may play a similar role for explicitly acquired procedural memory (e.g., Ref. 691 , see also sect. IV A ).
Infants and children sleep longer than adults. However, compared with adults, the proportion of REM sleep in children increases only during the first 6 mo of life, whereas the proportion of SWS remains enhanced throughout development until adolescence ( 480 , 866 ). The EEG during SWS also shows typical changes during development: the amplitude and slope of slow waves as well as SWA (i.e., spectral power in the 0.5–4 Hz band, including both <1 Hz slow oscillation and 1–4 Hz delta activity) increases until the beginning of puberty at the age of 10–12 years to levels distinctly higher than in adults, and thereafter starts to decrease ( 169 , 604 , 680 ). Several studies indicated that sleep disturbances and sleep loss impairs learning and school performance in children (for reviews, see Refs. 44 , 246 , 286 , 662 , 1178 ). Considering the importance of SWS specifically for the consolidation of hippocampus-dependent memory in adults, it was hypothesized that the effect of sleep on the consolidation of declarative memories is even greater during development ( 1321 ).
1. Consolidation of declarative memory
Backhaus et al. ( 56 ) reported beneficial effects of sleep on the consolidation of declarative memories (word pairs) in 9- to 12-yr-old children. The children's recall performance after sleep was not only better than that after the wake-retention intervals, but also compared with recall performance tested before sleep, suggesting a genuine gain of declarative memory that is produced by intervening sleep in children. Retention of word pairs across the sleep interval was positively correlated with the time spent in non-REM sleep and negatively with the time in REM sleep. Similarly, several other studies demonstrated profits from sleep in children between 7 and 14 years of age for word memories as well as for the integration of novel words into lexical knowledge ( 141 , 530 , 945 ). Similarly, in 7- and 12-yr-old children, memory for novel words profited from an off-line consolidation interval involving sleep ( 141 ). Sleep in children also preferentially enhanced emotional declarative memories (recognition of emotional pictures), whereas no effect of sleep on procedural memory consolidation (mirror tracing) was observed ( 947 ). A comparison of healthy children (10–16 years) with age-matched children with attention deficit/hyperactivity disorder (ADHD) revealed a superior sleep-dependent benefit for the retention of pictures in the healthy children ( 948 ). Although time in SWS did not differ between the groups, a significant positive correlation between slow oscillation power during non-REM sleep was only observed in the healthy children, possibly reflecting impaired functionality of this rhythm in ADHD children. In 14- to 16-yr-old adolescents, the restriction of sleep (to up to 5 h for 4 consecutive nights) did not impair either retention of word pairs or procedural memories for mirror tracing skill ( 1259 ). However, the adolescents showed a remarkable increase in the proportion of SWS during the restriction period, suggesting a high capability of the adolescent's brain to compensate effects of sleep restriction by flexibly increasing the sleep depth.
A beneficial effect of sleep in children (6–8 years) was found also for the consolidation of visuospatial memories in a two-dimensional object-location task known to involve the hippocampal function ( 1318 ). Unexpectedly, in this study comprising a comparison of memory performance in adults, the size of the sleep effect was closely comparable between children and adults, although the amount of SWS during experimental nights was on average more than twofold higher in the children. However, the direct comparison of retention rates for declarative materials between children and adults is generally hampered by the fact that consolidation can be sped up depending on the availability of associative schemas in long-term memory that can integrate new declarative information ( 1210 , 1211 ). In the adult brain, more of such schemas may be available than in the child's brain. Thus, irrespective of this confound, these studies overall point towards a particular relevance of the high amounts of SWS and associated SWA for declarative memory consolidation during development.
2. Consolidation of procedural memory
In contrast to the strong beneficial effects of non-REM sleep on the consolidation of declarative memory in children, studies examining procedural memories consistently demonstrated that in children, unlike in adults, posttraining sleep does not produce a robust improvement of skill. This finding is surprising considering that the acquisition of skills like learning to walk and to speak, to write, and to ride a bicycle represent major challenges of childhood. Also, the neuroanatomic structures underlying skill learning mature quite early during development, i.e., within the first 3 years of life ( 180 , 468 ). In fact, the majority of studies on sleep-dependent procedural memory formation in children indicated an impairing rather than improving effect of sleep on skill ( 385 , 947 , 949 , 1318 ), remarkably similar to findings in juvenile song birds where overnight sleep likewise deteriorated performance on the tutored song ( 280 ). In the respective studies, the sleep-dependent overnight gains in skill were measured with reference to performance before sleep rather than with reference to performance changes across a corresponding wake interval, which excludes that the absent gain of skill across sleep in children is due to a relatively greater capability in children to stabilize memories during wakefulness ( 318 ). Interestingly, sleep restored daytime deficits in procedural memory (serial reaction time task) in children with attention-deficit hyperactivity disorder, while again no overnight improvements were observed in healthy children ( 949 ).
One factor explaining the missing overnight gain in skill in children might be their fairly low initial skill levels. In adults, the level of skill performance at learning has repeatedly been demonstrated to modulate sleep-dependent memory consolidation, whereby benefits from sleep appeared to be most robust with intermediate performance levels ( 16 , 297 , 679 , 1151 ). In children, skill performance is generally much slower, less accurate, and less automated than in adults, particularly in the initial stage of training ( 318 , 385 , 1185 ), which gives rise to the question of whether sleep enhances skill memories also in children if presleep performance is improved to a level comparable with that in adults. Indeed, children (aged 4–6 years) who received high amounts of training in a finger-tapping task did develop a significant gain in tapping skill across sleep, whereas no such gain was observed in low performing children who had received the standard amount of training ( 1320 ). In the adults of this study, only the group with minimum training showed a consistent benefit from the nap. In combination, the findings in children and adults suggest that across age groups, the likelihood that sleep produces a distinct gain in skill performance is greatest when presleep performance is at an intermediate level. Owing to their generally quite low performance of skills, children reach levels of strength at which sleep-dependent benefits directly translate into benefits in behavioral speed and accuracy only after extensive levels of training.
3. Children extract more explicit knowledge from implicitly trained tasks than adults
Alternatively, the lack of sleep-dependent gains in skill performance in children might be ascribed to a competitive interaction between declarative and procedural memory systems ( 385 , 1318 ). Particularly in the initial stages of training, contributions of explicit (i.e., conscious) learning mechanisms involving prefrontal-hippocampal circuitry can be essential for skill acquisition, with the parallel storing of procedural and hippocampus-dependent declarative aspects favoring interactions and competitions between the two memory systems ( 16 , 43 , 937 , 1042 ), which can result in an impaired implicit task performance (e.g., slowing of reaction times) ( 1064 , 1135 ). Importantly, this competitive interaction between the memory systems might extend to processes of consolidation during sleep ( 143 , 147 , 273 , 1000 ). On the basis of the assumption that SWS predominantly supports consolidation of hippocampus-dependent declarative memory, it was argued that sleep in children, because of its great amounts of SWS, preferentially strengthens hippocampus-dependent explicit aspects in a skill representation, thereby hampering implicit performance. A recent study indeed confirmed a striking superiority of sleep in 8- to 11-yr-old children, compared with adult's sleep, to extract explicit declarative sequence knowledge from a coarse motor SRTT that was trained under implicit conditions (i.e., without being aware of the underlying sequence) before sleep ( 1322 ). On the generation task after retention sleep requiring the subjects to deliberately generate the eight-element sequence of cue positions underlying the SRTT trained before sleep, the children clearly outperformed the adults. The children's performance was also distinctly better than in control children who performed the generation task after training before the sleep interval. Superior explicit knowledge after sleep correlated with the amount of SWA in both age groups, in line with studies in adults indicating that SWS is critically involved in the extraction of explicit knowledge ( 1347 ).
Consistent with the notion that sleep in children particularly benefits explicit task aspects and the extraction of abstract knowledge, 15-mo-old infants already showed a sleep-dependent benefit for extraction of grammatical rules during language learning ( 469 ). The results were confirmed in a second study of this group employing an extended retention interval of 24 h ( 589 ). Both studies used the infant's orienting response towards the auditory word-strings to assess memory recall. Orienting is a well-known hippocampus-dependent function ( 1119 ), suggesting that the retrieval test tapped hippocampus-dependent declarative aspects of the memory, although this issue deserves further examination.
Together, the findings of a facilitating effect of sleep during development on the generation of hippocampus-dependent explicit knowledge about sequences and grammatical rules are well in line with the assumption of an active system consolidation established during sleep ( 293 , 1321 , 1327 ). According to this concept, processes during SWS supporting the redistribution of memories from temporary hippocampal to extrahippocampal long-term storage sites, do not only strengthen these memory representations, but also bring about qualitative transformations, manifesting themselves behaviorally in increased knowledge about invariant repeating and relevant features in the learned materials. Due to increased SWS in children, such transformations could be stronger during development than during adulthood.
C. Sleep and Memory Formation in Aged Rats and Humans
Sleep undergoes characteristic changes in the course of aging. Older adults sleep less and awaken more frequently during the night. Beginning already around the age of 40, there is a distinct decrease in SWS ( 167 , 866 ). Non-REM sleep in the elderly is characterized by decreases in SWA, especially over the prefrontal cortex, as well as decreases in spindle density ( 176 , 177 , 690 , 786 ). The time in REM sleep, on the other hand, remains relatively unchanged in late life. However, there is a significant decrease in the density of phasic REMs in elderly persons ( 254 ).
1. Hippocampus-dependent declarative memory
Considering that the major age-related changes in sleep architecture concern SWS and associated SWA, prominent impairments in the consolidation, especially of hippocampus-dependent declarative memory, may be expected ( 512 ). Consistent with this notion, aging rats at rest after spatial memory encoding showed weaker reactivations of temporal firing patterns in hippocampal neuron ensembles, and this was associated with diminished spatial memory performance in the Morris Water maze at the final testing day (e.g., Ref. 448 , see sect. III A4 ). The weakening of temporally sequenced reactivation patterns in aged rates did not appear to be a consequence of age-related decreases in SWS. Interestingly, simple reactivation patterns in CA1 cell ensembles, not taking into account the relative temporal order of cell-pair firing, was comparable between aged and young rats ( 449 ).
In humans, comparing retention of word pairs across nocturnal sleep between young (18–25 years) and middle-aged adults (48–55 years), Backhaus et al. ( 55 ) revealed a significantly lower retention of word pairs in the group of middle-aged participants. The impairment was observed specifically for a retention interval that covered early nocturnal sleep, which is typically dominated by SWS. SWS in this interval was strikingly lower in the middle-aged than young subjects. Also, percentages of time spent in SWS, but not in REM sleep, were strongly correlated with later retention performance. Overall, these data strongly suggest that the age-related deficits in declarative memory consolidation during sleep are due to a decline in SWS. In line with these findings, in aged individuals (69–80 years), the improvement in the recollection of hippocampus-dependent episodic memories (for personally experienced events) after retention periods of nocturnal sleep, with reference to recollection after daytime wakefulness, was less pronounced than in young (19–29 years) subjects ( 21 ). A recent study reported no beneficial effect of sleep on memory and no correlation between SWS and consolidation of word pairs across sleep in elderly subjects (60–84 years), whereas a positive correlation with SWS and a sleep-dependent memory improvement was observed in younger participants (18–22 years) ( 1055 ) A diminished capability to consolidate hippocampus-dependent memory (for word pairs) was not confirmed in a recent study by Wilson et al. ( 1324 ) including, besides young subjects, middle-aged (30–55 years) as well as old (55–70 years) participants. With reference to retention rates across daytime wake intervals, sleep improved retention rates in all age groups to the same extent. Although overall the findings point towards a diminished sleep-dependent consolidation of declarative memory in the aged due to deficits in SWS, the direct comparison of benefits from sleep between age groups might be confounded by the fact that age also diminishes memory retention across wakefulness, which is typically used as reference in these studies.
2. Procedural memory
With regard to procedural memory, several studies relying on different versions of the SRTT revealed age-related impairments in the formation of motor skill memories during sleep. Comparing older (45–80 years) and younger adults (18–24 years) on the SRTT under implicit and explicit learning conditions, Spencer et al. ( 1127 ) found that only the younger but not the older subjects distinctly improved in performance in both task versions across overnight sleep, compared with performance changes across a daytime retention period of wakefulness. Another study revealed sleep-dependent performance gains on a deterministic SRTT in middle-aged (35–55 years), but not in 55–70 years old participants ( 1324 ). In contrast, using a finger sequence tapping task, one study reported that elderly subjects (60–79 years) maintained their performance across 24 h including sleep, while their performance was impaired after 12 h of wakefulness, suggesting a role for sleep in optimal motor consolidation also in the elderly ( 1214 ). However, the overnight improvements typically observed in young subjects did not occur in these elderly participants. Sleep also did not benefit the performance of older (50–75 years) subjects on a continuous motor tracking task where the moving target to be tracked could follow a repeated sequence or move randomly similar to the SRTT ( 1079 – 1081 ). Interestingly, age-matched stroke patients in this study did show an improvement in tracing accuracy selectively across the sleep retention interval for both explicit and implicit performance conditions. Finally, sleep also did not improve performance in a second-order probabilistic SRTT in old subjects (60–80 years) ( 852 ). However, sleep-dependent benefits are also not consistently revealed in younger subjects for this task, possibly due to the great complexity of the underlying sequence rules associated with a higher degree of implicitness at learning ( 852 , 1122 , 1123 ).
To what extent the age-related decreases in sleep benefits for skill learning are related to specific alteration in sleep, in particular of SWS, is presently unclear as most of the relevant studies in aged subjects did not include polysomnographic recordings. REM sleep does not appear to be involved as neither experimental REM sleep deprivation nor REM sleep augmentation (i.e., the rebound after deprivation) produced any significant performance change in aged subjects on a procedural mirror tracing task ( 570 ). Nevertheless, like in young adults, high cholinergic activation during REM sleep seems to contribute to sleep-dependent consolidation of procedural memory also in aged individuals, as administration of the acetylcholinesterase inhibitor donezipil in aged subjects, together with an increase in REM density, significantly enhanced sleep-dependent gains in mirror tracing performance ( 570 , 961 ). The contributions of impaired SWS to the age-related decline in procedural memory consolidation remain to be specified in future studies.
VIII. SLEEP-DEPENDENT MEMORY CONSOLIDATION IN THE IMMUNE SYSTEM
A. memory formation in the immune system.
The immune system forms long-lasting memories for an antigen in a multistep process. The mounting of a so-called adaptive immune response is at the core of this process. Once antigens invade the organism at certain barriers, they are taken up and processed by antigen presenting cells (APC). APC carry the antigenic information to secondary lymphoid organs where they present fragments of the processed antigen to naive T cells, which become only activated if they express the specific cognate receptor that can recognize this particular antigenic epitope. The T cell recognizing its antigen, together with the APC, form the so-called immunological synapse. Activation of the T cell is linked to the release of different proinflammatory cytokines by APC, among which interleukin-12 (IL-12) is central for the subsequent differentiation of the activated T cell into T-helper 1 (Th1) cells. T-cell growth, proliferation, and differentiation is additionally supported by the release of IL-2, whereas the release of IL-10 in this context plays an antagonistic role favoring differentiation of Th2 cells and the recruitment of immediate but preformed humoral immune responses. Th1 cells also support the differentiation of B cells and their production of antigen-specific antibodies. Whereas most effector cells differentiated during acute infection do not survive for a long time after the infection, there is a subset of differentiated T and B cells that maintains the antigenic memory for the long-term. These memory cells mediate a speedy and more effective immune response upon reencounter of the antigen ( 13 , 1258 ).
There are apparent differences between memory formation in the immune system and in the central nervous system. Most obvious, in the immune system cells migrate to act in different tissues and body compartments and, unlike neurons, they often show strong proliferation in response to (antigenic) stimulation. These conditions may partly explain why memory formation in the immune system takes much longer (several days to weeks) than in the central nervous system. Nevertheless, immunological memory, like neurobehavioral memory, is formed as part of an adaptive organismic response to an environmental stressor, and shares the basic subprocesses of encoding, consolidation, and retrieval ( 641 , 695 ). Also, consolidation includes a cell-cell interaction in which the antigenic information is transferred from a temporary to a long-term store, with both stores represented by different cellular networks. Specifically, in this conceptual approach (see FIGURE 9 A ), encoding in the immune system would denote the uptake of antigenic information by APC. Consolidation would refer to processes at the immunological synapse that is subsequently formed between APC and T cells ( 338 , 473 ) and comprise the transfer of the antigenic information from the APC, only serving as temporary store, to the T cells serving as long-term store. Recall would refer to the faster and facilitated immune response when the antigen is re-encountered.

Sleep supports the initiation of an adaptive immune response. A : concept: the invading antigen is taken up and processed by antigen presenting cells (APC) which present fragments of the antigen to T helper (Th) cells, with the two kinds of cells forming an “immunological synapse.” The concomitant release of interleukin (IL)-12 by APC induces a Th1 response that supports the function of antigen-specific cytotoxic T cells and initiates production of antibodies by B cells. This response finally generates long-lasting immunological memory for the antigen. Sleep, in particular slow wave sleep (SWS), and the circadian system act in concert to generate a proinflammatory hormonal milieu with enhanced growth hormone and prolactin release and reduced levels of the anti-inflammatory stress hormone cortisol. The hormonal changes in turn support the early steps in the generation of an adaptive immune response in the lymph nodes. In analogy to neurobehavioral memory formed in the central nervous system, the different phases of immunological memory might be divided in an encoding, a consolidation, and a recall phase. In both the central nervous system and the immune system, sleep specifically supports the consolidation stage of the respective memory types. [Modified from Besedovsky et al. ( 90 ).] B : sleep enhances the hepatitis A virus (HAV)-specific T helper (Th) cell response to vaccination (three shots at weeks 0, 8, and 16, vertical syringes) in two groups of human subjects who either slept (black circle, thick line) or stayed awake (white circle, thin line) in the night following inoculations. The immune response as indicated by the frequency of CD40L+ HAV-specific Th cells (percentage of total Th cells) at weeks 18–20 ( left panel ) and particularly at week 52 ( right panel ) is strongly predicted by the amount of slow wave activity (averaged across the three postinoculation nights). Values are means ± SE: * P ≤ 0.1; ** P ≤ 0.05; *** P ≤ 0.01. [Data from Lange et al. ( 694 ).]
B. Effects of Sleep on the Formation of an Adaptive Immune Response
1. experimental vaccination.
Vaccination as an experimental model of infection has been used as a straightforward approach to comprehensively assess effects of sleep on the formation of immunological memory mainly in humans where this approach quite consistently revealed an enhancing effect of sleep on the adaptive immune response and measures of antigenic memory. Human volunteers who slept normally in the first night after a single vaccination against hepatitis A, 4 weeks after the vaccination displayed a twofold increase in antigen-specific antibody titers, compared with participants who stayed awake on the first night after vaccination ( 697 ). Similar effects were found in recent experiments using repeated and combined inoculations with hepatitis A and B antigens ( FIGURE 9 B , Ref. 694 ). In this study, nocturnal sleep after the inoculations doubled blood counts of antigen-specific Th cells, as marker of antigenic memory. During antigen reencounter, these Th cells stimulate the production of antibodies specifically directed against hepatitis A and B. Moreover, sleep profoundly increased the proportion of T cells producing proinflammatory and Th1-cytokines like IL-2, interferon-γ (IFN-γ), and tumor necrosis factor-α (TNF-α). The effects persisted over 1 year. In a further study, short sleep duration after hepatitis B vaccination predicted a decreased antibody response to the immunization ( 946 ). Together, these studies provide a compelling demonstration that nocturnal sleep after vaccination suffices to strengthen the evolvement of an adaptive, i.e., memory-forming immune response to a clinically relevant extent. A strengthening role of sleep for immunological memory formation is also supported by observations of reduced total IgG under conditions of sleep restriction (to 4 h/night) for several days after vaccination against influenza ( 1130 ).
In contrast to studies in humans, which examined effects of sleep on primary immune response developing to the first encounter of an antigen, studies in animals have so far concentrated on secondary immune responses, i.e., the effect of sleep on the recall of an antigenic memory that was already established during a preceding primary response. Overall, these studies revealed inconsistent results with sleep, compared with conditions of sleep deprivation, enhancing or weakening markers of recall, or showing no effect ( 145 , 980 , 981 , 1206 ). Because of their focus on secondary immune responses, these animal studies basically cannot be compared with the studies of primary responses in humans.
The strengthening effects of sleep on the human primary immune response to infection were revealed with sleep occurring within the first 36 h after inoculation. This time window suggests that sleep affects the cascade of responses to the viral challenge at a rather early stage. Indeed, research has identified two main targets that are affected by sleep in this process, i.e., 1 ) the interactions between APC and T cells at the immunological synapse and 2 ) the migration of these cells to secondary lymphoid tissues.
2. Sleep supports APC-T cell interactions
There is converging evidence that sleep promotes Th1 immune responses as an immediate sequel of APC-T cell interactions. Thus sleep in humans enhances the production of IL-12 by monocytes and premyolid dendritic cells (pre-mDC) which represent the most important precursors of mature professional APC circulating in blood ( 307 , 696 ). Concurrently, sleep diminishes the production of anti-inflammatory IL-10 by monocytes ( 696 ). Furthermore, several reports indicated an enhancing influence of sleep on T-cell production of IL-2 which acts to further promote T-cell growth, proliferation, and differentiation ( 120 , 595 ), although other studies failed to reveal such effects when examining IL-2 production in specific T-cell subpopulations ( 109 , 308 ). Sleep increased proliferation of Th cells as well as the activity of natural regulatory T (nTreg) cells ( 109 ). Concurrent upregulation of nTreg activity might reflect a counterregulatory response preventing overshooting of Th1 responses. Moreover, early nocturnal sleep increased the ratio in IFN-γ/IL-4 production by Th cells, indicating a shift in the Th1/Th2 cytokine balance towards preponderant production of Th1 cytokines ( 308 ). In patients with allergic rhinitis, wheal reactions to a skin prick test and spontaneous IgE production of peripheral blood mononuclear cells were increased after a night of total sleep deprivation, indicating a shift from Th1 to prevailing Th2 immune defense ( 637 ). Finally, there is evidence that sleep increases blood concentrations of IL-7, a cytokine fostering differentiation of T memory cells ( 76 ). Together, these findings speak for the notion that sleep specifically benefits APC-T cell interactions towards the development of predominant Th1 immune responses.
3. Effects of sleep on the migration of T cells and APC to lymphoid tissues
Sleep appears to affect also the migratory pattern of leukocytes, although the trafficking of T cells as well as APC has not been studied thoroughly in the context of sleep. Cells centrally involved in the formation of adaptive immunity, like naive and central memory T cells, show a circadian rhythm with the highest numbers of circulating cells at night in humans. This circadian rhythm is mainly driven by cortisol which upregulates the chemokine receptor CXCR4 on these cells and thereby facilitates their redistribution to the bone marrow during daytime ( 305 , 693 ). On top of this circadian rhythm, nocturnal sleep, compared with wakefulness, induces a slight but significant reduction in circulating naive and central memory T cells ( 695 ), which indeed might reflect a redistribution of these cells to lymph nodes that is specific to sleep. In rodents, counts of lymphocytes as well as percentages of Th cells showed a variation across the 24-h period that ran in parallel in blood, spleen, and lymph nodes, with peak counts reached during the rest period, suggesting a quick redistribution of T cells from the vascular compartment to secondary lymphatic tissues. Two studies, one in sheep ( 289 ) and the other in humans ( 351 ), provided more direct evidence that the efflux of lymphocytes from lymph nodes is reduced during sleep. In rats, lymphocyte counts in lymph nodes indeed increased after recovery sleep that followed a period of sleep deprivation ( 1354 ). The mechanisms underlying this sleep-induced homing of T cells to lymph nodes are not clear. Homing to the lymph node via high endothelial venules relies on adhesion molecules and chemokine receptors (CD62L, CCR7, and CD11a) expressed on the T-cell membrane. In addition to the suppression of cortisol release during early nocturnal SWS, sleep may ease T-cell trafficking to lymph nodes via sympathetic and peptidergic innervation of lymph nodes and influences on cytokine release ( 117 , 234 , 876 ).
In combination, these data support the view that sleep favors an accumulation of T cells in lymph nodes, and there might be a parallel effect on APC migration ( 307 , 627 ). These migratory effects probably occur in concert with synergistic actions of the circadian rhythm and eventually might serve to enhance the rate of APC-Th cell interactions in secondary lymphoid tissues during sleep. In contrast to sleep, wakefulness appears to support the recruitment of cells in circulating blood that have high cytotoxic effector potential, like proinflammatory monocytes, terminally differentiated cytotoxic T cells, and cytotoxic natural killer (NK) cells, and serve the immediate immune defense against pathogens invading the organism preferentially during the activity period (e.g., Refs. 42 , 287 ).
4. Contribution of SWS to memory formation in the immune system
Although effects of the experimental manipulation of specific sleep stages on the adaptive immune response have not, thus far, been systematically assessed, there is converging though indirect evidence that the strengthening effects of sleep on the formation of long-term antigenic memory originates from processes during SWS. The sleep-induced increase in antigen-specific Th cells after hepatitis A vaccination was strongly correlated with the amount SWS and EEG SWA on the night after vaccination ( 693 ). The increase in percentage of hepatitis A virus specific antigen-specific Th cells measured 18–20 wk after vaccination was correlated with r = 0.72, and that measured 1 year later with a coefficient of r = 0.94 with SWA. Other measures, including REM sleep-related parameters, were not predictive of this increase. Corresponding findings were obtained in rabbits, where the amount of SWS predicted the survival rate after a pathogen challenge ( 1205 ). Moreover, in humans, early nocturnal SWS-rich sleep is associated with a shift towards Th1 cytokine activity predominating over Th2 activity which is replaced by a shift towards the predominance of Th2 cytokine production during late sleep when SWS is fading and REM sleep becomes prevalent ( 308 ). In fact, peaks in Th1 cytokine activity and, more generally, in proinflammatory cytokine activity during the rest period were mainly detected during the early SWS-dominated portion of sleep, in humans as well as in animals in different tissues including the brain ( 487 , 671 ) and lymph nodes ( 359 ), but also in blood and circulating leukocytes ( 109 , 120 , 172 , 306 , 308 , 487 , 696 , 749 , 1158 ). Interestingly, some of these cytokines were found, conversely, to promote SWS, pointing towards a positive-feedback loop between the central nervous and immune systems ( 693 ).
5. Endocrine mediation of immunological memory formation during sleep
The strengthening effect of sleep on immunological memory formation has been ascribed to the endocrine milieu as it is generated specifically during SWS. In humans, early nocturnal SWS is associated with an increased release of the immune-supportive hormones prolactin and growth hormones while release of the immunosuppressive glucocorticoids is suppressed ( 117 ). Sleep, specifically SWS, contributes to the generation of this unique endocrine pattern in concert with circadian mechanisms ( 91 , 1125 , 1126 , 1129 ). Not only SWS, but also the enhanced release of GH and prolactin, together with the simultaneously suppressed cortisol levels observed during SWS-rich sleep after hepatitis A vaccination, were revealed to be highly predictive for the number of antigen-specific Th cells detected in blood 4 wk and 1 year after vaccination ( 696 ). In fact, blocking of mineralocorticoid receptors during early SWS-rich sleep produces an enhancement in naive T-helper cell counts ( 89 ). Moreover, in vivo as well as in vitro studies identified high prolactin and low cortisol levels as factors most strongly contributing to the nocturnal upsurge of IL-12 production ( 307 , 696 ). GH and prolactin are well known to promote T-cell proliferation and differentiation as well as Th1 cytokine activity ( 193 , 217 , 436 , 814 ), and to develop adjuvant-like actions when given shortly after vaccination ( 1124 , 1136 ). Low glucocorticoid levels during SWS-rich sleep contribute to these effects as these hormones are highly potent anti-inflammatory agents suppressing proinflammatory and Th1 cytokine activity ( 693 ). Probably, other factors (orexin, leptin, autonomic innervation of lymphoid tissues, etc.) add to this mediation of sleep effects, but these are as of yet entirely unexplored. Overall, these findings corroborate the view that during SWS, which dominates the early night, an endocrine milieu is established that supports the initiation of an adaptive immune response as a basis for the formation of long-lasting antigenic memory. Conversely, there are hints that owing to an overshooting proinflammatory cytokine response, the immediate defense of antigen is impaired during sleep, particularly during SWS ( 508 , 627 , 693 , 779 ).
It is important to note here that sleep, and particularly SWS, has been likewise identified as a factor critically involved in the formation of long-term memories in the neurobehavioral domain ( 292 ). These findings indicate that memory formation during sleep in both the immune and the central nervous system, beyond conceptual analogies, shares causal mechanisms. This, indeed, conveys the idea that forming long-term memory is a general function of sleep, which eventually serves to strategically adapt the organism to environmental stressors in entirely different domains.
IX. CONCLUDING REMARKS
For more than a century it has been known that memory benefits from sleep, and research in this field has put forward different explanations for this phenomenon. Here we aimed to establish a review covering the progress of research in this field of sleep and memory in its entireness, simultaneously taking into account the vastly differing approaches that have been adopted to clarify the mechanism mediating the memory benefit from sleep. Whereas initially it was commonly assumed that sleep improves memory in a passive manner, by protecting it from being overwritten by interfering external stimulus inputs, the current theorizing assumes an active consolidation of memories that is specifically established during sleep, and basically originates from the reactivation of newly encoded memory representations. In parallel, the perspective on the researched memory has changed. Whereas initial research largely concentrated on the stability of memory, demonstrating how sleep contributes to the persistence of a memory in all of its qualities, recent research has begun to concentrate on the dynamics of memory formation, systematically examining the changes a memory representation undergoes during sleep-dependent consolidation. The active system consolidation process assumed to take place during sleep leads to a transformation and a qualitative reorganization of the memory representation, whereby the “gist” is extracted from the newly encoded memory information and integrated into the long-term knowledge networks.
Most recently, the focus of research in the field has broadened, indicating that sleep benefits memory not only in the neurobehavioral domain, but also in the formation of immunological long-term memories, stimulating the idea that forming long-term memories represents a general function of sleep. There are first cues that sleep-dependent memory formation in the immune and central nervous system share common mechanisms, that in both domains appear to be linked to SWS. Also in the immune system, sleep appears to support the intercellular reorganization of memory representations such that during the APC-T cell interaction the epitopic information is extracted from the antigen to be stored by T cells. Certain features of active system consolidation, like cell assembly reactivation of neurobehavioral representations, can occur also during wakefulness, but with different consequences. The effective reorganization of the representation requires a specific milieu of neurotransmitter and endocrine activity as it is established only during sleep, specifically during SWS. Subsequent REM sleep may then be involved in strengthening the reactivated and reorganized representations on a molecular and synaptic level. Thus sleep and wakefulness appear to be associated with different and mutually exclusive modes of memory processing, with sleep favoring processes of memory consolidation that are incompatible with the efficient encoding and retrieval of stimuli, as required while coping with environmental demands in the wake phase.
This work was supported by Deutsche Forschungsgemeinschaft Grant SFB 654 Plasticity and Sleep and Swiss National Foundation Grant PP00P1_133685.
DISCLOSURES
No conflicts of interest, financial or otherwise, are declared by the authors.
ACKNOWLEDGMENTS
We thank Maren Cordi, Anja Otterbein, Ursula Rasch, Julia Rihm, Thomas Schreiner, Sarah Schoch, and Manuela Steinauer for assistance in preparing the manuscript and Sandra Ackermann-Wohlgemuth, Luciana Besedovsky, Susanne Diekelmann, and Ines Wilhelm for helpful comments on earlier versions of the manuscript. In particular, we thank Drs. Ted Abel, Igor Timofeev, and Paul Shaw for very constructive comments and corrections.
Addresses for reprint requests and other correspondence: J. Born, Institute of Medical Psychology and Behavioral Neurobiology, Univ. of Tuebingen, Gartenstraße 29, 72074 Tuebingen, Germany (e-mail: [email protected] ); or B. Rasch, Institute of Psychology, Div. of Biopsychology, Univ. of Zurich, Binzmühlestrasse 14, Box 5, CH-8050 Zurich Switzerland (e-mail: [email protected] ).
Shining a Light on the Mechanisms of Sleep for Memory Consolidation
- Sleep and Learning (M Scullin, Section Editor)
- Open access
- Published: 15 July 2021
- Volume 7 , pages 221–231, ( 2021 )
Cite this article
You have full access to this open access article
- Michelle A. Frazer 1 ,
- Yesenia Cabrera 1 ,
- Rockelle S. Guthrie 2 &
- Gina R. Poe ORCID: orcid.org/0000-0003-3388-2858 3
7626 Accesses
4 Citations
55 Altmetric
Explore all metrics
Purpose of review
This paper reviews all optogenetic studies that directly test various sleep states, traits, and circuit-level activity profiles for the consolidation of different learning tasks.
Recent findings
Inhibiting or exciting neurons involved either in the production of sleep states or in the encoding and consolidation of memories reveals sleep states and traits that are essential for memory. REM sleep, NREM sleep, and the N2 transition to REM (characterized by sleep spindles) are integral to memory consolidation. Neural activity during sharp-wave ripples, slow oscillations, theta waves, and spindles are the mediators of this process.
These studies lend strong support to the hypothesis that sleep is essential to the consolidation of memories from the hippocampus and the consolidation of motor learning which does not necessarily involve the hippocampus. Future research can further probe the types of memory dependent on sleep-related traits and on the neurotransmitters and neuromodulators required.
Similar content being viewed by others
When Counting Sheep Doesn’t Help: The Effects of Cocaine on Sleep
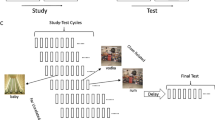
A complementary learning systems model of how sleep moderates retrieval practice effects
Xiaonan L. Liu, Charan Ranganath & Randall C. O’Reilly
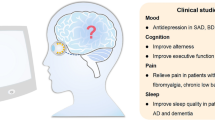

A Comprehensive Overview of the Neural Mechanisms of Light Therapy
Xiaodan Huang, Qian Tao & Chaoran Ren
Avoid common mistakes on your manuscript.
Introduction
Sleep is a period of heightened vulnerability for an organism—an extended stretch of time during which an animal is oblivious to its surroundings, cut off from environmental cues that would alert it to a potential food source, an approaching mate, or an impending danger. And yet, despite all of the potential survival-related drawbacks that such a state entails, every animal sleeps. From drosophila to laboratory rats to humans, evolution has conserved this unguarded state across time. Clearly, the function of sleep is important enough to outweigh the danger of being in such an unguarded state. Researchers have proposed a range of potential roles for each sleep state, including recovery from oxidative stress in the brain [ 1 , 2 ], energy conservation [ 3 ], a period of heightened protein synthesis [ 4 , 5 ], and the stimulation of a sleeping brain to facilitate transitions into waking [ 6 ]. A somewhat more controversial proposition, however, is that sleep is an important stage of time for the consolidation of memories created during waking.
Sleep is divided into multiple stages (Fig. 1a ); each of which is identified by stereotyped behavior, electrical activity, and neurochemical composition. The broadest distinction splits sleep into two stages, rapid eye movement (REM) and non REM (NREM) sleep. REM is characterized by muscle atonia, desynchronized waking-like EEG activity, and a 5–10 Hz hippocampal theta rhythm. NREM sleep displays 0–4 Hz slow oscillation / delta activity, accompanied by fast sharp-wave ripple (SWR) events in the hippocampus. Determining the role of each sleep stage and its signatures in the consolidation process is an area of intense investigation within the field of sleep research.
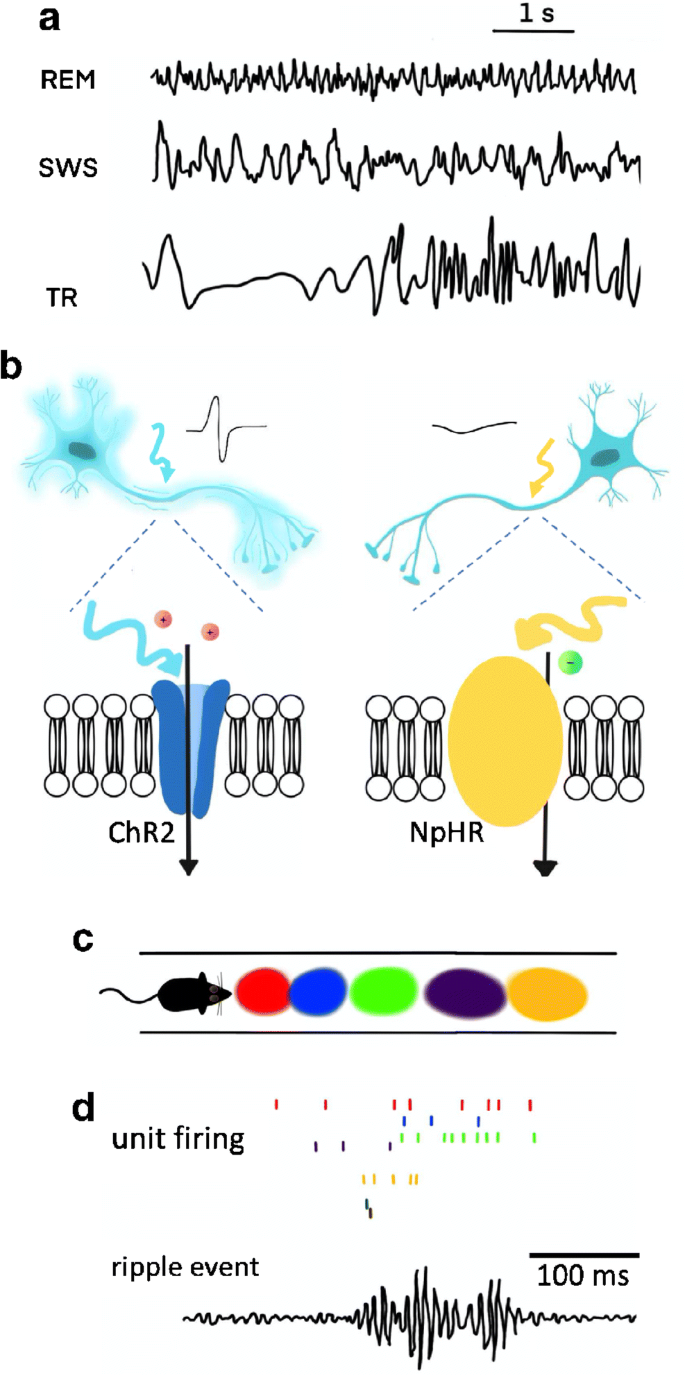
A ) Sample traces of rodent hippocampal EEG during sleep. Top: the 5-10 Hz theta of rapid eye movement (REM) sleep. Middle: Characteristic 2-4 Hz delta rhthym of slow wave sleep. Bottom: Examples of 10-14 Hz sigma in the form of sleep spindles that occur in brief periods of transition to REM (TR) sleep. B ) Light-activated channels inserted into the neuronal membrane regulating activity. Stimulating channels such as ChR2 allow for the influx of cations when activated via blue light, resulting in membrane depolarization and action potential generation. To inhibit neural activity, light-triggered ion pumps are typically used, such as NpHR, which pumps in CI- ions to hyperpolarize the membrane. C ). As an animal (left, from above) moves along its path, place cells (represented by different colors) encode the spatial context. D ) An example of hippocampal replay during SWS. During SWS, ripple events in the hippocampus are generated by unit, firing of different place cells that at high frequencies, often in the same order as places encountered during waking.
Experimentalists have been investigating the role of sleep in learning and memory storage for years, finding that depriving animals of normal sleep nearly always results in adverse outcomes in their ability to store and retain new information. For example, early studies of REM deprivation in rodents showed decreased performance on hippocampal-dependent working memory tasks, especially contextual/spatial mazes that require established cognitive maps of the animals’ environment [ 7 , 8 , 9 , 10 ]. Physiological studies indicate that REM deprivation results in a loss of excitability in the rodent hippocampus [ 11 , 12 ] and reduces long-term potentiation (LTP) [ 13 ]. Accumulated evidence demonstrates that NREM sleep is beneficial for declarative memory in humans and may provide a time for communication between disparate brain areas, particularly the hippocampus and prefrontal cortex through the generation of sleep spindles, which are correlated to memory recall [ 14 , 15 ]. Conversely, REM sleep may be an important period for learning procedural tasks [ 16 , 17 ].
While the body of evidence supporting an important role for sleep in memory consolidation grows, a number of critiques have plagued the field since its inception and proven themselves difficult to fully resolve. Limitations in the specificity of past methods as well as incongruous and conflicting study results have led some prominent sleep researchers to altogether reject any role for sleep in memory processing, instead viewing sleep solely through the lens of homeostatic regulation and systemic physiological function [ 6 , 18 , 19 ]. New techniques such as optogenetics enable researchers to target light-activated ion channels to control the activity of select brain areas, neural populations, or specific memory traces (Fig. 1b ). Using light-activated channels such as channelrhodopsin (ChR2) and halorhodopsin (NpHR) allows for precise spatial and temporal neural activation or inhibition in order to tease apart the function of specific structures and cell populations [ 20 ]. Modulating neurons at such a granular level during sleep can tell us how activity outside of waking encoding (offline) shapes the connections and circuits of a memory ensemble. In this review, we aim to look at the ways in which optogenetics has allowed us to more specifically delineate the role of sleep in the consolidation of waking experiences (Table 1 ). We find that optogenetic techniques enable the modulation of various facets of sleep, while avoiding many of the confounding variables obscuring the results of earlier studies.
Stress Is Not the Culprit: Sleep Feature Disruption Using Optogenetics Causes Learning and Memory Deficiencies Without the Stress of Behavioral Sleep Deprivation Methods
One of the biggest critiques of early sleep studies was the difficulty in dissociating sleep loss from the stress induced by methods of sleep deprivation as playing the causal role in subsequent learning deficits. Methods to disrupt REM sleep can result in hypothalamic-pituitary-adrenal (HPA) axis activation and increased cortisol levels, which have been shown to negatively impact hippocampal dependent memory consolidation [ 36 , 37 , 38 ]. These methods led researchers to argue that the behavioral deficits seen were a result of impaired hippocampal function due to stress rather than sleep loss. Indeed, stress does impair hippocampal neurogenesis just as chronic sleep disruption and sleep deprivation does, and perhaps the two mechanisms are the same [ 37 , 38 ]. With the use of optogenetics, the studies discussed herein target specific sleep stages to modulate sleep features in a manner that does not introduce stress as a confounding variable.
One set of experiments used an optogenetic protocol to fragment sleep without inducing stress in the animal [ 36 ]. By selectively expressing ChR2, an excitatory optogenetic ion channel (Fig. 1b ), in hypocretin/orexin (Hcrt) neurons in the lateral hypothalamus (LH), investigators were able to decrease latency to arousal in both REM and NREM, resulting in fragmented sleep periods. Stimulation of the LH at 60 s intervals with blue light resulted in Hrct neurons firing action potentials and was enough to fragment sleep, as measured by microarousal periods. Overall sleep amount, quality, and composition of sleep was unchanged, and no evidence of sleep debt occurred following the stimulation protocol, indicating that only the continuity of sleep was disrupted. Recent research suggests that this arousal effect might be mediated through extensive projections to the locus coeruleus (LC), an important structure for sleep and arousal regulation [ 39 , 40 , 41 ]. To ensure that the animal was not under stress due to stimulation, investigators tested cortisol levels in the plasma of both ChR2 and control mice following the 4-h stimulation protocol, finding no difference between stimulated and unstimulated animals. Similarly, animals that underwent the optogenetic stimulation did not show increased anxiety behavior in an open-field maze. Taken together, these findings show that the optogenetically induced sleep fragmentation protocol did not increase physiological markers of stress or behavioral indications of increased anxiety.
The Hcrt stimulation-induced sleep disruption paradigm allowed researchers to directly test the effect of discontinuous sleep on learning and memory. Using the novel object recognition (NOR) task as a measure of hippocampal- and sleep-dependent memory [ 42 , 43 , 44 ], they ran animals through the optogenetic stimulation protocol during sleep in the crucial 4 h window during the light period immediately following training on the task [ 9 ]. In animals with Hcrt neurons expressing ChR2, the blue light stimulation resulted in a significant impairment in NOR performance as compared to control animals. Importantly, this effect was only seen when stimulation occurred in the light period following task acquisition, not in the dark period hours later (outside the critical consolidation window), highlighting the role of Hcrt neurons in sleep-dependent consolidation rather than a result of aberrant Hcrt activity alone. Additionally, this effect was abolished when stimulation intervals were increased to 120 s apart, indicating that there is a minimum length (> 60 s) of sleep “quanta” necessary for adequate memory consolidation in the rodent. Another study found that 60-s long inductions of SWS repeatedly for 30 min within 30 min of learning stabilized the memory such that discrimination was maintained for as long as 6 h after object place exposure and novel arm Y maze task [ 27 ]. They produced SWS by optogenetically stimulating the GABAergic neurons in the parafacial zone, thereby increasing delta and reducing EMG activity as is consistent with the induction of SWS. The 60-s long inductions of SWS also stabilized contextual fear memory and improved context discrimination in these animals.
It would be interesting to know what that minimum undisturbed sleep quanta for memory stabilization and consolidation is in the human. Even as few as 5 airway obstruction–related arousals per hour (equating to once every 12 min) is associated with memory deficits in humans [ 45 ].
The ability to separate the effects of sleep deprivation from the methods used to sleep deprive and the negative effects they can cause allows research to move toward understanding the role that each component of sleep plays in the memory consolidation process. Work in our lab has used optogenetics to change baseline activity in neuromodulatory systems during sleep without disrupting overall time spent in sleep or sleep architecture itself [ 26 •]. Unlike the Hcrt study, we did not induce arousals from sleep, yet found similar memory consolidation deficits related to changes in the electrophysiological features of each sleep state. We optogenetically modulated the locus coeruleus (LC) activity during sleep, and provided correlational evidence for the role various sleep signatures play in sleep-dependent consolidation. Typically, the LC quiets during NREM periods and is silenced during REM sleep [ 46 ]. Maintaining LC activity at waking levels throughout sleep after learning resulted in a decrease in the 1–4 Hz delta band and in the 10–14 Hz sigma band corresponding to sleep spindles. Longer spindles, which increased in control learning animals, were nearly eliminated when LC activity was maintained during sleep. In REM sleep, theta power was significantly decreased during LC stimulation. These changes were correlated with poor performance in incorporating changes made in a spatial memory task. Greater reductions in delta and theta band power were directly related to an increase in switching away from reliance on the hippocampal map. Similarly, reductions in spindle-ripple coupling predicted confusion between the original and modified food positions on the maze. The ability to adjust the frequency of LC activation with optogenetics during sleep allowed us to keep normal sleep bout lengths, yet still observe changes in memory consolidation.
The fact that changing the underlying physiology of sleep without disrupting other aspects of sleep affects memory consolidation suggests that the brain activity associated with each sleep state, and the particular neurochemical composition associated with each phase of sleep, is critical in supporting memory consolidation. Additional studies have found that optogenetic modulation of neuronal subtypes during sleep can similarly disrupt memory consolidation. Researchers investigating the inhibition of parvalbumin interneurons (PV+) during NREM sleep found that this intervention abolished the typical post-learning sleep increase in delta and theta power in the hippocampus [ 28 ]. In another group of animals that were sleep deprived, a similar decrease in delta and theta power was observed. These delta and theta power decreases were concurrent with disrupted contextual fear memory in both groups. However, activating hippocampal parvalbumin interneurons rhythmically at theta frequency during sleep deprivation stabilized CA1 population activity measured during subsequent sleep and rescued appropriate post-sleep hippocampus-dependent fear behavior. These findings suggest the necessity of sleep at least partially lies in the population activity engendered during the state and provides evidence that this activity directs the consolidation of memories.
Optogenetics Allow Us to Target and Modulate Specific Sleep Signatures, to Test Their Causal Relationship to Learning and Memory
The phenomenon of hippocampal replay present in NREM sleep has long intrigued sleep researchers as a possible mechanism by which labile memory traces in the hippocampus are stabilized and sent to the neocortex for long-term storage. Replay consists of cells active during waking task acquisition (Fig. 1c ) being reactivated during sleep (Fig. 1d ) with conserved temporal order. Ripples (Fig. 1d ) take place in the CA1 region of the hippocampus indicating fast, synchronous excitatory inputs from the principal cells of the CA3. Replay of waking sequences occurs at the peak of ripples [ 47 , 48 ]. Recruitment into ripple activity is at least in part associated with the strength of connections between cells formed during wakefulness—cells that are significantly more likely to fire together during waking are more likely to fire together during ripple associated replay events [ 47 , 49 ]. Thus cell participation in ripple events is heavily biased by waking patterns of activity. These findings served as the basis for the theory that SWR and replay activity therein are mechanisms for strengthening and storing memories.
Indeed, when SWR activity is electrically disrupted during sleep following acquisition of a new hippocampus-dependent task, a significant impairment is observed in an animal’s ability to perform those tasks, likely due to aberrant consolidation [ 50 , 51 ]. Whether this performance impairment was due to the loss of SWRs specifically, or simply the disruption of hippocampal population activity due to the electrical stimulation, was not testable until optogenetics became a common technique.
Studies utilizing optogenetics found that selectively inhibiting pyramidal cells of the CA1 once SWRs were detected resulted in a significant decrease in an animal’s ability to reinstate assembly activity representing a novel environment [ 34 ]. Intriguingly, inhibiting SWRs did not result in a change in assembly activity representing already consolidated familiar environments, suggesting the necessity of hippocampal SWR activity for consolidating new information. In addition, novel assemblies that stabilized more rapidly than others during waking acquisition were also unaffected by the disruption of offline reactivation, perhaps because their original online activity was enough to result in synaptic stabilization, obviating the necessity of consolidation. These findings support the hypothesis that offline activity is important for resolving unfinished business, strengthening weak connections made during waking.
A second study using a similar paradigm in which principal CA1 cells express an inhibitory optogenetic ion channel investigated the effect of SWR suppression during the 3 h following passive exploration of a novel open field. They explored the ability of hippocampal place cells to subsequently encode the same open field environment after inhibiting pyramidal activity for 500 ms triggered after the detection of the ripple. No explicit learning task was given for the animals in the environment. Surprisingly, no significant differences were found between the place cell stability, coherence, or likelihood of remapping in the novel environment between animals with and without CA1 pyramidal activity during spontaneous SWR in this offline period [ 32 ] . Given the task impairment seen in Girardeau et al. [ 50 ], as well as the change in assembly activity in novel environments of the van de Ven [ 34 ] paper, one might conclude that disrupting the place cell code during the consolidation of novel memories underlies the spatial memory deficits seen following SWR inhibition. On the surface, this conclusion is not born out by Kovacs et al. [ 32 ] . However, they did observe a post-inhibition rebound in pyramidal cell activation that appeared to be coincident with strong local field potential (LFP) depolarization and, in their example, occurred in the ripple frequency. The apparent rebound depolarization-coupled ripple activity could itself have stabilized the memory code. Also, the authors point out that passive exploration may be relatively resistant to memory disruption by SWR inhibition as compared with active learning. For instance, a task in which learning is involved (and tested), such as an appetitive maze, should require the encoding and association of more information than is required when wandering in an open field. Another possibility is that ripples are involved primarily in the transmission of information to long-term cortical storage, and their disruption does not have an effect on spatial map stabilization within the hippocampus. Future experiments should be able to distinguish between these possibilities.
The question of whether REM sleep has a role in learning and memory has been particularly controversial [ 6 , 18 ]. In rodents, early sleep deprivation studies indicated that REM sleep is necessary for proper memory formation; however, as discussed above, the methods available at the time came with numerous caveats, leaving some sleep researchers skeptical of whether REM deprivation was truly causing memory impairments. Similar to our ability to optogenetically disrupt sleep as a whole, we now can design experiments selectively targeting REM sleep independently to isolate its role in memory processing without causing stress to the animal or affecting other sleep states. What we have learned about REM sleep thus far suggests that it serves several key functions in consolidation.
REM Sleep Is for Forgetting
REM sleep appears to be a time during which synapses are pruned, creating circuits with more sparse and more specific firing. Recent studies in mice show that the pruning of dendritic spines in the motor cortex (M1) during REM sleep is coincident with the improvement on a rotarod task [ 52 , 53 ]. Similarly, the firing of adult-born neurons (ABNs) in the dentate gyrus becomes more sparse in response to contextual fear conditioning (CFC) following a period of REM sleep [ 21 •]. These data provide evidence that one of the functions of REM sleep is to pare down excessive activity following learning, and suggests a role for REM sleep in “forgetting” or weakening memories.
Studies of melanin-concentrating hormone (MCH) neurons in the hypothalamus provide further evidence for the function of REM in forgetting [ 24 ]. Optogenetically inhibiting REM-active MCH neurons during REM periods following the learning phase of a novel object recognition (NOR) task improves the animals’ ability to discriminate between new and familiar objects. The converse was also true—when MCH cells were optogenetically activated during REM periods, the animals’ ability to discriminate between novel and familiar objects was diminished, as was their performance in the second task: contextual memory following fear conditioning. As this study also found that REM periods normally involve MCH cell activity, REM sleep appears to play a role in forgetting. It may be that the normal MCH activity during REM serves the function of schematic integration and extraction of gist, losing details as memories are moved to long-term storage or schematized.
REM Sleep Is for Remembering
To complicate matters further, however, evidence also exists that REM sleep promotes consolidation as well. The inhibition of theta activity by silencing medial septal GABAergic projections to the hippocampus during REM sleep significantly disrupted consolidation of CFC and novel object place recognition (NOPR) tasks [ 31 ]. This result raises the possibility that REM sleep is a time for both strengthening and weakening synapses due to the unique electrophysiological and neurochemical properties of the hippocampus in REM sleep. Hippocampal theta activity during REM sleep segregates the population activity into temporal cycles [ 54 , 55 , 56 , 57 ], which may be key to allowing both synaptic strengthening and weakening during this period of time. Previous studies from our lab show that spatial representations fire differently in relation to theta phase based on their novelty or familiarity [ 58 ]. New place cells fire at peak population activity, resulting in synaptic strengthening, while place cells encoding familiar spaces fire closer to the low point of activity, likely weakening the associated synapses through the principles of heterosynaptic depotentiation [ 58 ]. In addition to the theta rhythm, the neurochemical composition of the hippocampus during REM sleep, particularly the complete lack of noradrenaline and high levels of acetylcholine, could make it an optimal time for restructuring synaptic connections and integrating new information into old memory schema [ 25 •, 59 ].
Population Activity and Timing Is Key in Sleep-Dependent Consolidation
Through the temporal precision afforded by optogenetic techniques, sleep researchers have been able to tease apart the function of various sleep signatures and oscillatory activity. What we are finding suggests that the effect that a particular sleep stage or signature has on a neural ensemble is highly dependent on the population activity surrounding the ensemble. We have a great deal of evidence that reactivation of an ensemble during sleep strengthens the circuitry involved [ 47 , 48 ]; however, it was initially unclear if this strengthening was simply a result of activity-dependent plasticity that could occur during any brain state. Furthermore, activity within sleep stages must be tightly temporally regulated in order to properly consolidate information. Studies suggest that the timing of action potentials of cells in an ensemble in relation to oscillatory population activity is critical to whether the connections of a memory trace will be strengthened or weakened. In a series of studies utilizing a brain-machine interface task in rats, researchers determined that whether an ensemble reactivated in closer proximity to a slow oscillation (SO) or to a delta wave had an effect on whether the memory was consolidated or weakened [ 29 ]. Ultimately, they found that perturbing spiking activity during the upstates of SOs led to an impairment of memory consolidation, whereas disrupting spiking during the upstate of a delta wave actually boosted sleep-dependent memory consolidation. Further exploration suggests that one underlying mechanism of the changes in consolidation is dependent on nesting of spindles within SOs or delta waves. SO-spindle coupling appears to preserve memory reactivation, as reactivation strength and duration increased with SO-spindle coupling, and changes to SO-spindle coupling correlated with task performance. Interestingly, when researchers optogenetically perturbed SO spiking activity, a rapid reduction in reactivation strength followed. The converse was true when delta activity was disrupted, resulting in significantly stronger and longer-lasting reactivation, supporting the idea that inhibiting delta waves increased the strength of a memory. Ultimately, inhibiting SO activity abolished any significant rescaling of the neural network involved in the task used, resulting in impaired performance. In another study, this group inhibited the specific neural ensemble controlling the task selectively during the upstates of NREM sleep, which also impaired task performance as well as the ability of the ensemble to develop sparse coding of the task [ 22 •]. Finally, another group showed the same relationship between SO-spindle coupling and spindle-nested ripples that indicate cell activation. When they increased sleep spindles by activating parvalbumin interneurons in the reticular nucleus of the thalamus, they increased spindle-ripple coupling in the upstate of SO’s and increased memory on both contextual fear memory and novel-object place recognition [ 30 •]. These data all support the hypothesis that sleep is a time during which synaptic connections are both strengthened and weakened in order to efficiently and accurately encode an animal’s environment.
Neural Ensembles Require Offline Processing to Accurately Consolidate New Information
Optogenetic techniques allow for further exploration into the circuit-level changes underlying disrupted memory consolidation following abnormal sleep dependent activity. Alteration of sleep state activity described in the experiments above broadly results in memory impairment suggesting a relationship between processing during sleep and integrity of memory ensembles. Although they provide invaluable insights, direct manipulation of neural ensemble activity during sleep is required.
Rhythmic Activity like the Theta Oscillation Is Important for Memory Consolidation
So far, studies suggest that ensembles undergo reshaping during offline processing that can lead to both strengthening and weakening of synaptic connections. The participation of these ensembles in the local rhythmic activity present in sleep appears to be crucial for proper memory formation, in a manner that is highly specific. In an experiment by Kumar et al., optogenetically modulating the activity of adult-born neurons (ABNs) elucidated the role of these cells in consolidating contextual fear memories during REM sleep [ 21 •]. Their study shows that the tuning of ABNs representing the CFC memory during REM sleep is critical to the animal’s ability to recall this memory the following day. Inactivating the ABN ensemble during REM sleep resulted in poor consolidation of the CFC memory, suggesting the necessity of ABN activity in the DG during REM sleep for memory retention. Silencing ABN activity during NREM sleep following learning did not produce the same effect, indicating a role for REM activity of ABNs in the processing of fear memories.
Non-ensemble Cells Are Not Involved
Intriguingly, when ABNs that were not part of the memory encoding process were randomly stimulated during REM sleep, consolidation was also impaired. This suggests that the integrity of the ensemble active during REM processing is critical to proper consolidation. Similarly, it provides evidence of the causal nature of sleep reactivation for memory consolidation—it suggests a role for the unique population activity of sleep stages in shaping ensemble circuits, which directly contributes to the retention of a memory.
Cortical Ensembles Also Participate in Offline Processing for Memory Consolidation
The necessity of ensemble reactivation during sleep appears to be critical to brain regions outside of the hippocampus as well. When looking at CFC in the retrosplenial cortex (RSC), researchers found a similarly important role for reactivation in learning and memory [ 22 •]. Isolating stimulation to cells active during a CFC task enabled tightly controlled temporal and spatial modulation of the ensemble presumed to represent the CFC memory trace. To examine the necessity of activation during offline processing to memory integrity, researchers optogenetically stimulated the fear memory ensemble during both anesthesia and natural sleep; ultimately, both conditions produced similar results. This study in particular focused on examining the potential role of sleep-dependent processing in transferring memories to long-term storage. Predictably, animals with activity in their hippocampi blocked 24 h after learning did not form the proper association between the context and the shock, indicating an improperly consolidated fear memory. This was not the case in the animals that underwent optogenetic stimulation of the RSC memory trace during either anesthesia or sleep right after learning—these mice displayed normal freezing behavior despite the 24 h later hippocampal block, suggesting that high-frequency activation of the trace ensemble was able to create a hippocampus-independent memory of the fear trace, effectively accelerating the process of systems consolidation. Intriguingly, the optogenetic stimulation protocol was only effective when the animals were in a non-waking state. Stimulation of the fear trace while animals were awake and behaving did not protect against memory impairment following hippocampal block, indicating that sleep state activity, or anesthesia plus artificially stimulated activity, is necessary for systems consolidation. Which aspects of sleep are crucial for this process, however, are uncertain, as these experiments did not differentiate between REM or NREM sleep. Additionally, as the researchers note, their stimulation protocol did not maintain the temporal patterns of activity. Given the difference in effectiveness of the optogenetic stimulation between wakefulness and sleep, future research into whether stimulation during only REM or NREM is still effective in inducing systems consolidation would provide more evidence into the role of each sleep stage in long-term memory storage.
Similar work in the visual cortex assessed the effect of offline stimulation of the neural ensemble on memory consolidation [ 60 •]. Rhythmically activating this trace while an animal was anesthetized biased surrounding neurons toward responding to the same cue during subsequent waking, suggesting a role for offline activity in modifying responsiveness of visual cortical neurons to allow visual discrimination. Inhibition of the cue ensemble during REM and NREM sleep resulted in a generalized behavioral response to all gradients. It will be interesting to observe in future studies what, if anything, distinguishes the roles of REM and NREM sleep in consolidation.
Conclusions and Future Directions
In summary, applying optogenetic techniques to sleep research has provided an exciting new avenue by which to understand the mechanisms involved in sleep-dependent memory consolidation. We are now able to say with confidence that the negative effects of sleep deprivation on memory consolidation are not solely due to the anxiety- and stress-inducing methods of sleep deprivation. Instead, we have begun to determine the specific functions of sleep signatures such as ripples coupled with spindles nested in slow oscillations and theta. The studies we covered here included demonstrations of sleep’s involvement in hippocampus-dependent learning such as spatial learning and contextual fear learning, perceptual learning-like visual pattern and texture discrimination learning, motor learning, and even neuroprosthetic learning, the consolidation of all of which are disrupted by specific sleep feature manipulations: delta, theta, spindles, spindle-ripple coupling, and slow oscillation spindle coupling during REM and non-REM sleep. In fact, using optogenetics to selectively disrupt these features of sleep without altering overall sleep architecture or structure consistently finds learning and memory disruptions, unlike the gross sleep deprivation or disruption studies which have produced more variable results.
Despite these advances, the mysterious nature of sleep still remains, as do challenges to the sleep-memory hypothesis. For example, we are still unsure of the degree to which experimental sleep studies done in rodents (where the vast majority of our knowledge of sleep’s electrophysiology comes from) are applicable to humans. Because of the difficulty of obtaining human intracranial electrophysiological data, for example, we cannot be fully confident that sleep signatures important to rodent learning and memory, such as hippocampal replay or REM sleep theta, are present in human sleep, let alone critical for our sleep-dependent processing. In order to link sleep disturbances to disease and psychiatric illness, more studies will need to be done to demonstrate that sleep-dependent consolidation processes in laboratory animal models are analogous to human sleep. Without the ability to target specific neural ensembles in the human brain, as is possible when using optogenetics in animal models, researchers instead use targeted memory reactivation (TMR) as a method to modulate electrophysiological activity during sleep. TMR involves the re-exposure of sensory cues (odor or sound) during sleep that were present during wakeful learning. Unlike the use of light in rodent optogenetic studies, TMR uses cue presentations as a method to selectively reactivate neural ensembles associated with the stimulus. Since the discovery that TMR modulates neural activity in the sleeping brain at the time of cue presentation and improves memory of associated items at subsequent testing by Rasch et al. [ 61 ], researchers have sought to elucidate to what extent findings in rodent studies apply to humans. For a full review of recent publications related to this topic, see Cellini and Mednick 2018 [ 62 ] and Mankin and Fried 2020 [ 63 ]. The use of intracranial recordings to explore sleep in select patient populations as well as new non-invasive technology such as magnetoencephalography in healthy participants will help shed light on some of these questions.
The power of optogenetics as a technique comes from allowing researchers to design experiments that target neurons for modulation in a temporally and spatially specific manner. For sleep research in particular, this means being better equipped to test the underlying mechanisms of sleep without adding confounding variables such as stress or sleep architecture changes into experiments. From just the last few years of research into sleep using optogenetics, we have gathered convincing evidence that without sleep, memories are not processed correctly, largely due to the disturbance of activity patterns underlying sleep signatures such as ripples and spindles. We are beginning to see evidence of sleep-dependent processing occurring in various regions of the brain such as the visual cortex and retrosplenial cortex and, through optogenetic interruption, learning that when the electrophysiology of sleep is disrupted, memory consolidation is impaired as well. The specificity allowed by optogenetic techniques means we are able to narrow down our inquiries to single neuronal ensembles representing a memory trace and observe the impact of sleep-dependent consolidation on that memory alone.
Papers of particular interest, published recently, have been highlighted as: • Of importance •• Of major importance
Ramanathan L, Gulyani S, Nienhuis R, Siegel JM. Sleep deprivation decreases superoxide dismutase activity in rat hippocampus and brainstem. NeuroReport. 2002;13:1387–90.
Article CAS Google Scholar
Gopalakrishnan A, Ji LL, Cirelli C. Sleep Deprivation and cellular responses to oxidative stress. Sleep. 2004;27:27–35. https://doi.org/10.1093/sleep/27.1.27 .
Article PubMed Google Scholar
Lima SL, Rattenborg NC, Lesku JA, Amlaner CJ. Sleeping under the risk of predation. Anim Behav. 2005;70:723–36. https://doi.org/10.1016/j.anbehav.2005.01.008 .
Article Google Scholar
Seibt J, Dumoulin MC, Aton SJ, Coleman T, Watson A, Naidoo N, et al. Protein synthesis during sleep consolidates cortical plasticity in vivo. Curr Biol. 2012;22:676–82. https://doi.org/10.1016/j.cub.2012.02.016 .
Article CAS PubMed PubMed Central Google Scholar
Ramm P, Smith CT. Rates of cerebral protein synthesis are linked to slow wave sleep in the rat. Physiol Behav. 1990;48:749–53. https://doi.org/10.1016/0031-9384(90)90220-X .
Article CAS PubMed Google Scholar
Vertes RP, Eastman KE. The case against memory consolidation in REM sleep. Behav Brain Sci. 2000;23:867–76. https://doi.org/10.1017/S0140525X00004003 .
Youngblood BD, Zhou J, Smagin GN, Ryan DH, Harris RBS. Sleep deprivation by the “flower pot” technique and spatial reference memory. Physiol Behav. 1997;61:249–56. https://doi.org/10.1016/S0031-9384(96)00363-0 .
Youngblood BD, Smagin GN, Elkins PD, Ryan DH, Harris RBS. The effects of paradoxical sleep deprivation and valine on spatial learning and brain 5-HT Metabolism. Physiol Behav. 1999;67:643–9. https://doi.org/10.1016/S0031-9384(99)00120-1 .
Smith C, Rose GM. Evidence for a paradoxical sleep window for place learning in the Morris water maze. Physiol Behav. 1996;59:93–7. https://doi.org/10.1016/0031-9384(95)02054-3 .
Smith C, Conway J, Rose G. Brief paradoxical sleep deprivation impairs reference, but not working, memory in the radial arm maze task. Neurobiol Learn Mem. 1998;69:211–7. https://doi.org/10.1006/nlme.1997.3809 .
McDermott CM, LaHoste GJ, Chen C, Musto A, Bazan NG, Magee JC. Sleep deprivation Causes behavioral, synaptic, and membrane excitability alterations in hippocampal neurons. J Neurosci. 2003;23:9687–95. https://doi.org/10.1523/JNEUROSCI.23-29-09687.2003 .
McDermott CM, Hardy MN, Bazan NG, Magee JC. Sleep deprivation-induced alterations in excitatory synaptic transmission in the CA1 region of the rat hippocampus. J Physiol. 2006;570:553–65. https://doi.org/10.1113/jphysiol.2005.093781 .
Ribeiro S, Mello CV, Velho T, Gardner TJ, Jarvis ED, Pavlides C. Induction of hippocampal long-term potentiation during waking leads to increased extrahippocampal zif-268 expression during ensuing rapid-eye-movement sleep. J Neurosci. 2002;22:10914–23. https://doi.org/10.1523/JNEUROSCI.22-24-10914.2002 .
Schabus M, Gruber G, Parapatics S, Sauter C, Klösch G, Anderer P, et al. Sleep spindles and their significance for declarative memory consolidation. Sleep. 2004;27:1479–85. https://doi.org/10.1093/sleep/27.7.1479 .
Demanuele C, Bartsch U, Baran B, Khan S, Vangel MG, Cox R, et al. Coordination of slow waves with sleep spindles predicts sleep-dependent memory consolidation in schizophrenia. Sleep. 2017;40. https://doi.org/10.1093/sleep/zsw013 .
Mednick S, Nakayama K, Stickgold R. Sleep-dependent learning: a nap is as good as a night. Nat Neurosci. 2003;6:697–8. https://doi.org/10.1038/nn1078 .
Karni A, Tanne D, Rubenstein BS, Askenasy JJ, Sagi D. Dependence on REM sleep of overnight improvement of a perceptual skill. Science. 1994;265:679–82. https://doi.org/10.1126/science.8036518 .
Siegel JM. The REM sleep-memory consolidation hypothesis. Science. 2001;294:1058–63. https://doi.org/10.1126/science.1063049 .
Vertes RP, Siegel JM. Time for the sleep community to take a critical look at the purported role of sleep in memory processing. Sleep. 2005;28:1228–9. https://doi.org/10.1093/sleep/28.10.1228 .
Pastrana E. Optogenetics: controlling cell function with light. Nat Methods. 2011;8:24–5. https://doi.org/10.1038/nmeth.f.323 .
Kumar D, Koyanagi I, Carrier-Ruiz A, Vergara P, Srinivasan S, Sugaya Y, et al. Sparse activity of hippocampal adult-born neurons during REM sleep is necessary for memory consolidation. Neuron. 2020;(107):552, e10–65. https://doi.org/10.1016/j.neuron.2020.05.008 Adult-born neurons in the dentate gyrus are critical for forming contextual fear memories. REM periods following contextual fear conditioning pare down the activity of these neurons, and silencing them during REM sleep results in unsuccessful fear memory consolidation .
de Sousa AF, Cowansage KK, Zutshi I, Cardozo LM, Yoo EJ, Leutgeb S, et al. Optogenetic reactivation of memory ensembles in the retrosplenial cortex induces systems consolidation. Proc Natl Acad Sci. 2019;116:8576–81. https://doi.org/10.1073/pnas.1818432116 Reactivating neural ensembles outside of the hippocampus is also shown to result in systems consolidation .
Davis CJ, Vanderheyden WM. Optogenetic sleep enhancement improves fear-associated memory processing following trauma exposure in rats. Sci Rep . 2020;10:18025. https://doi.org/10.1038/s41598-020-75237-9 .
Izawa S, Chowdhury S, Miyazaki T, Mukai Y, Ono D, Inoue R, et al. REM sleep–active MCH neurons are involved in forgetting hippocampus-dependent memories. Science. 2019;365:1308–13. https://doi.org/10.1126/science.aax9238 .
Kim J, Gulati T, Ganguly K. Competing roles of slow oscillations and delta waves in memory consolidation versus forgetting. Cell. 2019;179:514–526.e13. https://doi.org/10.1016/j.cell.2019.08.040 Inhibiting the neural ensemble in the motor cortex during the more global slow oscillation upstates impairs memory consolidation, while driving more spindle-slow oscillation upstate coupling through motor neuron ensemble inhibition during the more local delta wave upstates enhances sleep-dependent memory motor learning consolidation .
Swift KM, Gross BA, Frazer MA, Bauer DS, KJD C, Vazey EM, et al. Abnormal Locus coeruleus sleep activity alters sleep signatures of memory consolidation and impairs place cell stability and spatial memory. Curr Biol. 2018;28:3599–3609.e4. https://doi.org/10.1016/j.cub.2018.09.054 Eliminating LC silences during sleep results in unstable spatial representations and performance deficits on a spatial memory task that correlate with delta and theta rhythm amplitude reductions, with curtailment of longer spindles, and with disruptions in spindle-ripple timing that implicate different memory consolidation functions for each sleep state .
Lu Y, Zhu ZG, Ma QQ, Su YT, Han Y, Wang X, et al. A critical time-window for the selective induction of hippocampal memory consolidation by a brief episode of slow-wave sleep. Neurosci Bull. 2018;34:1091–9. https://doi.org/10.1007/s12264-018-0303-x .
Ognjanovski N, Broussard C, Zochowski M, Aton SJ. Hippocampal network oscillations rescue memory consolidation deficits caused by sleep loss. Cereb Cortex. 2018;28:3711–23. https://doi.org/10.1093/cercor/bhy174 .
Article PubMed PubMed Central Google Scholar
Gulati T, Guo L, Ramanathan DS, Bodepudi A, Ganguly K. Neural reactivations during sleep determine network credit assignment. Nat Neurosci. 2017;20:1277–84. https://doi.org/10.1038/nn.4601 .
Latchoumane CV, Ngo HV, Born J, Shin HS. Thalamic spindles promote memory formation during sleep through triple phase-locking of cortical, thalamic, and hippocampal rhythms. Neuron. 2017;95(2):424–435.e6 https://pubmed.ncbi.nlm.nih.gov/28689981/ . Timing optogenetic spindle induction to slow oscillation up-phase, along with co-occurring ripples, directly contributes to fear memory consolidation .
Boyce R, Glasgow SD, Williams S, Adamantidis A. Causal evidence for the role of REM sleep theta rhythm in contextual memory consolidation. Science. 2016;352:812–6. https://doi.org/10.1126/science.aad5252 .
Kovács KA, O’Neill J, Schoenenberger P, Penttonen M, Guerrero DKR, Csicsvari J. Optogenetically Blocking sharp wave ripple events in sleep does not interfere with the formation of stable spatial representation in the CA1 area of the hippocampus. PLoS One. 2016;11:e0164675. https://doi.org/10.1371/journal.pone.0164675 .
Miyamoto D, Hirai D, Fung CCA, Inutsuka A, Odagwa M, Suzuki T, et al. Top-down cortical input during NREM sleep consolidates perceptual memory. Science. 2016:1315–8 https://science.sciencemag.org/content/352/6291/1315.full .
van de Ven GM, Trouche S, McNamara CG, Allen K, Dupret D. Hippocampal offline reactivation consolidates recently formed cell assembly patterns during sharp wave-ripples. Neuron. 2016;92:968–74. https://doi.org/10.1016/j.neuron.2016.10.020 .
McNamara CG, Tejero-Cantero Á, Trouche S, Campo-Urriza N, Dupret D. Dopaminergic neurons promote hippocampal reactivation and spatial memory persistence. Nat Neurosci. 2014;17(12):1658–60 https://pubmed.ncbi.nlm.nih.gov/25326690/ .
Rolls A, Colas D, Adamantidis A, Carter M, Lanre-Amos T, Heller HC, et al. Optogenetic disruption of sleep continuity impairs memory consolidation. Proc Natl Acad Sci. 2011;108:13305–10. https://doi.org/10.1073/pnas.1015633108 .
Guzman-Marin R, Suntsova N, Methippara M, Greiffenstein R, Szymusiak R, McGinty D. Sleep deprivation suppresses neurogenesis in the adult hippocampus of rats. Eur J Neurosci. 2005;22:2111–6. https://doi.org/10.1111/j.1460-9568.2005.04376.x .
Mirescu C, Peters JD, Noiman L, Gould E. Sleep deprivation inhibits adult neurogenesis in the hippocampus by elevating glucocorticoids. Proc Natl Acad Sci. 2006;103:19170–5. https://doi.org/10.1073/pnas.0608644103 .
Bourgin P, Huitrón-Reséndiz S, Spier AD, Fabre V, Morte B, Criado JR, et al. Hypocretin-1 modulates rapid eye movement sleep through activation of locus coeruleus neurons. J Neurosci. 2000;20:7760–5. https://doi.org/10.1523/JNEUROSCI.20-20-07760.2000 .
Carter ME, de Lecea L, Adamantidis A. Functional wiring of hypocretin and LC-NE neurons: implications for arousal. Front Behav Neurosci. 2013;7. https://doi.org/10.3389/fnbeh.2013.00043 .
España RA, Reis KM, Valentino RJ, Berridge CW. Organization of hypocretin/orexin efferents to locus coeruleus and basal forebrain arousal-related structures. J Comp Neurol. 2005;481:160–78. https://doi.org/10.1002/cne.20369 .
Palchykova S, Winsky-Sommerer R, Meerlo P, Dürr R, Tobler I. Sleep deprivation impairs object recognition in mice. Neurobiol Learn Mem. 2006;85:263–71. https://doi.org/10.1016/j.nlm.2005.11.005 .
Chen L, Tian S, Ke J. Rapid eye movement sleep deprivation disrupts consolidation but not reconsolidation of novel object recognition memory in rats. Neurosci Lett. 2014;563:12–6. https://doi.org/10.1016/j.neulet.2014.01.024 .
Cohen SJ, Munchow AH, Rios LM, Zhang G, Ásgeirsdóttir HN, Stackman RW. The rodent hippocampus is essential for nonspatial object memory. Curr Biol. 2013;23:1685–90. https://doi.org/10.1016/j.cub.2013.07.002 .
Corinna K, Dieter R, Nofzinger EA, Bernd F, Josef U, Ruth O’H, et al. Memory before and after sleep in patients with moderate obstructive sleep apnea. J Clin Sleep Med. 2009;05:540–8. https://doi.org/10.5664/jcsm.27655 .
Aston-Jones G, Bloom FE. Activity of norepinephrine-containing locus coeruleus neurons in behaving rats anticipates fluctuations in the sleep-waking cycle. J Neurosci. 1981;1:876–86. https://doi.org/10.1523/JNEUROSCI.01-08-00876.1981 .
Wilson MA, McNaughton BL. Reactivation of hippocampal ensemble memories during sleep. Science. 1994;265:676–9. https://doi.org/10.1126/science.8036517 .
Kudrimoti HS, Barnes CA, McNaughton BL. Reactivation of hippocampal cell assemblies: effects of behavioral state, experience, and EEG dynamics. J Neurosci. 1999;19:4090–101. https://doi.org/10.1523/JNEUROSCI.19-10-04090.1999 .
Pavlides C, Winson J. Influences of hippocampal place cell firing in the awake state on the activity of these cells during subsequent sleep episodes. J Neurosci. 1989;9:2907–18. https://doi.org/10.1523/JNEUROSCI.09-08-02907.1989 .
Girardeau G, Benchenane K, Wiener SI, Buzsáki G, Zugaro MB. Selective suppression of hippocampal ripples impairs spatial memory. Nat Neurosci. 2009;12:1222–3. https://doi.org/10.1038/nn.2384 .
Ego-Stengel V, Wilson MA. Disruption of ripple-associated hippocampal activity during rest impairs spatial learning in the rat. Hippocampus. 2010;20:1–10. https://doi.org/10.1002/hipo.20707 .
Li W, Ma L, Yang G, Gan W-B. REM sleep selectively prunes and maintains new synapses in development and learning. Nat Neurosci. 2017;20:427–37. https://doi.org/10.1038/nn.4479 .
Zhou Y, Lai CSW, Bai Y, Li W, Zhao R, Yang G, et al. REM sleep promotes experience-dependent dendritic spine elimination in the mouse cortex. Nat Commun. 2020;11:4819. https://doi.org/10.1038/s41467-020-18592-5 .
Fernández-Ruiz A, Oliva A, Nagy GA, Maurer AP, Berényi A, Buzsáki G. Entorhinal-CA3 dual-input control of spike timing in the hippocampus by theta-gamma coupling. Neuron. 2017;93:1213–1226.e5. https://doi.org/10.1016/j.neuron.2017.02.017 .
Dragoi G, Buzsáki G. Temporal encoding of place sequences by hippocampal cell assemblies. Neuron. 2006;50:145–57. https://doi.org/10.1016/j.neuron.2006.02.023 .
Foster DJ, Wilson MA. Hippocampal theta sequences. Hippocampus. 2007;17:1093–9. https://doi.org/10.1002/hipo.20345 .
Gupta AS, van der Meer MAA, Touretzky DS, Redish AD. Segmentation of spatial experience by hippocampal theta sequences. Nat Neurosci. 2012;15:1032–9. https://doi.org/10.1038/nn.3138 .
Poe GR, Nitz DA, McNaughton BL, Barnes CA. Experience-dependent phase-reversal of hippocampal neuron firing during REM sleep. Brain Res. 2000;855:176–80. https://doi.org/10.1016/S0006-8993(99)02310-0 .
Poe GR. Sleep Is for Forgetting. J Neurosci. 2017;37:464–73. https://doi.org/10.1523/JNEUROSCI.0820-16.2017 .
Clawson BC, Pickup EJ, Enseng A, Geneseo L, Shaver J, Gonzalez-Amoretti J, et al. Causal role for sleep-dependent reactivation of learning-activated sensory ensembles for fear memory consolidation. Nat Commun. 2021;12:1200. https://doi.org/10.1038/s41467-021-21471-2 Visual cue-responsive neurons are selectively reactivated during post-fear conditioning sleep. When these ensembles are inhibited, fear memory consolidation is disrupted, suggesting the selective reactivation of sensory information during sleep is necessary for memory consolidation .
Rasch B, Büchel C, Gais S, Born J. Odor cues during slow-wave sleep prompt declarative memory consolidation. Science. 2007;315:1426–9 https://pubmed.ncbi.nlm.nih.gov/17347444/ .
Cellini N, Mednick SC. Stimulating the sleeping brain: current approaches to modulating memory-related sleep physiology. J Neurosci Methods. 2019;316:125–36 https://pubmed.ncbi.nlm.nih.gov/30452977/ .
Mankin EA, Fried I. Modulation of human memory by deep brain stimulation of the entorhinal-hippocampal circuitry. Neuron. 2020;106(2):218–35. https://doi.org/10.1016/j.neuron.2020.02.024 .
Download references
Acknowledgements
Supported by NIMH 60-670. YC was supported by a Cota-Robles Fellowship. RSG was supported by the Integrative Biology and Physiology Eureka Scholarship and T32 NS115753. We thank Rockelle Guthrie for helpful suggestions and for initiating and populating much of the table.
Author information
Authors and affiliations.
Brain Research Institute Neuroscience Interdepartmental Program, University of California, Los Angeles, Los Angeles, CA, USA
Michelle A. Frazer & Yesenia Cabrera
Department of Integrative Biology and Physiology, Molecular, Cellular, and Integrative Physiology Program, Program, University of California, Los Angeles, CA, 90095, USA
Rockelle S. Guthrie
The Department of Integrative Biology and Physiology, and Psychiatry and Biobehavioral Sciences, University of California, Los Angeles, 610 Charles E. Young Dr. E, Los Angeles, CA, 90095, USA
Gina R. Poe
You can also search for this author in PubMed Google Scholar
Contributions
MAF wrote the ms and prepared the figure and citations. YC co-wrote the ms and contributed to the table. RSG made the table and contributed to the ms. GRP co-wrote the ms and edited the figure and table.
Corresponding author
Correspondence to Gina R. Poe .
Ethics declarations
Conflicts of interest.
The authors declare no competing interests.
Human and Animal Rights and Informed Consent
This review article does not report any previously unpublished studies with human or animal subjects performed by any of the authors.
Additional information
Publisher’s note.
Springer Nature remains neutral with regard to jurisdictional claims in published maps and institutional affiliations.
This article is part of the Topical Collection on Sleep and Learning
Rights and permissions
Open Access This article is licensed under a Creative Commons Attribution 4.0 International License, which permits use, sharing, adaptation, distribution and reproduction in any medium or format, as long as you give appropriate credit to the original author(s) and the source, provide a link to the Creative Commons licence, and indicate if changes were made. The images or other third party material in this article are included in the article's Creative Commons licence, unless indicated otherwise in a credit line to the material. If material is not included in the article's Creative Commons licence and your intended use is not permitted by statutory regulation or exceeds the permitted use, you will need to obtain permission directly from the copyright holder. To view a copy of this licence, visit http://creativecommons.org/licenses/by/4.0/ .
Reprints and permissions
About this article
Frazer, M.A., Cabrera, Y., Guthrie, R.S. et al. Shining a Light on the Mechanisms of Sleep for Memory Consolidation. Curr Sleep Medicine Rep 7 , 221–231 (2021). https://doi.org/10.1007/s40675-021-00204-3
Download citation
Accepted : 23 March 2021
Published : 15 July 2021
Issue Date : December 2021
DOI : https://doi.org/10.1007/s40675-021-00204-3
Share this article
Anyone you share the following link with will be able to read this content:
Sorry, a shareable link is not currently available for this article.
Provided by the Springer Nature SharedIt content-sharing initiative
- Sharp-wave ripples
- Slow oscillations
- Depotentiation
- Optogenetics
- Consolidation
- Hippocampus
- Motor learning
- Spatial learning
Advertisement
- Find a journal
- Publish with us
- Track your research
- History, Facts & Figures
- YSM Dean & Deputy Deans
- YSM Administration
- Department Chairs
- YSM Executive Group
- YSM Board of Permanent Officers
- FAC Documents
- Current FAC Members
- Appointments & Promotions Committees
- Ad Hoc Committees and Working Groups
- Chair Searches
- Leadership Searches
- Organization Charts
- Faculty Demographic Data
- Professionalism Reporting Data
- 2022 Diversity Engagement Survey
- State of the School Archive
- Faculty Climate Survey: YSM Results
- Strategic Planning
- Mission Statement & Process
- Beyond Sterling Hall
- COVID-19 Series Workshops
- Previous Workshops
- Departments & Centers
- Find People
- Biomedical Data Science
- Health Equity
- Inflammation
- Neuroscience
- Global Health
- Diabetes and Metabolism
- Policies & Procedures
- Media Relations
- A to Z YSM Lab Websites
- A-Z Faculty List
- A-Z Staff List
- A to Z Abbreviations
- Dept. Diversity Vice Chairs & Champions
- Dean’s Advisory Council on Lesbian, Gay, Bisexual, Transgender, Queer and Intersex Affairs Website
- Minority Organization for Retention and Expansion Website
- Office for Women in Medicine and Science
- Committee on the Status of Women in Medicine Website
- Director of Scientist Diversity and Inclusion
- Diversity Supplements
- Frequently Asked Questions
- Recruitment
- By Department & Program
- News & Events
- Executive Committee
- Aperture: Women in Medicine
- Self-Reflection
- Portraits of Strength
- Mindful: Mental Health Through Art
- Event Photo Galleries
- Additional Support
- MD-PhD Program
- PA Online Program
- Joint MD Programs
- How to Apply
- Advanced Health Sciences Research
- Clinical Informatics & Data Science
- Clinical Investigation
- Medical Education
- Visiting Student Programs
- Special Programs & Student Opportunities
- Residency & Fellowship Programs
- Center for Med Ed
- Organizational Chart
- Faculty Affairs Department Teams
- Committee Info (Login Required)
- Recent Appointments & Promotions
- Academic Clinician Track
- Clinician Educator-Scholar Track
- Clinican-Scientist Track
- Investigator Track
- Traditional Track
- Research Ranks
- Instructor/Lecturer
- Social Work Ranks
- Voluntary Ranks
- Adjunct Ranks
- Other Appt Types
- Appointments
- Reappointments
- Transfer of Track
- Term Extensions
- Timeline for A&P Processes
- Interfolio Faculty Search
- Interfolio A&P Processes
- Yale CV Part 1 (CV1)
- Yale CV Part 2 (CV2)
- Samples of Scholarship
- Teaching Evaluations
- Letters of Evaluation
- Dept A&P Narrative
- A&P Voting
- Faculty Affairs Staff Pages
- OAPD Faculty Workshops
- Leadership & Development Seminars
- List of Faculty Mentors
- Incoming Faculty Orientation
- Faculty Onboarding
- Past YSM Award Recipients
- Past PA Award Recipients
- Past YM Award Recipients
- International Award Recipients
- Nominations Calendar
- OAPD Newsletter
- Fostering a Shared Vision of Professionalism
- Academic Integrity
- Addressing Professionalism Concerns
- Consultation Support for Chairs & Section Chiefs
- Policies & Codes of Conduct
- Health & Well-being
- First Fridays
- Fund for Physician-Scientist Mentorship
- Grant Library
- Grant Writing Course
- Mock Study Section
- Research Paper Writing
- Funding Opportunities
- Join Our Voluntary Faculty
- Faculty Resources
- Research by Keyword
- Research by Department
- Research by Global Location
- Translational Research
- Research Cores & Services
- Program for the Promotion of Interdisciplinary Team Science (POINTS)
- CEnR Steering Committee
- Experiential Learning Subcommittee
- Goals & Objectives
- Embryonic Stem Cell Research Oversight
- COVID-19 Vaccinations in CT
- COVID-19 in Connecticut Schools
- Connecticut Towns COVID-19 Impact Dashboard
- Connecticut Town 14-Day Cases Time Lapse
- CT Correctional Facilities with COVID Cases Dashboard
- Connecticut COVID Presence Map
- CT Nursing Homes with COVID-19 Cases
- U.S. COVID Presence Map
- COVID-19 Case Density by US County
- Global Cases Dashboard
- Time-Lapse of Global Spread
- US Racial and Ethnic Disparities in COVID-19 Mortality
- Childcare Survey and Data Display
- Risk of Complications Conditional on COVID-19 Infection
- Travel Time to COVID Testing Sites in Connecticut
- Travel Time to COVID Testing Sites in the US
- Project Team
- Issues List
- Print Magazine PDFs
- Print Newsletter PDFs
- YSM Events Newsletter
- Social Media
- Patient Care
INFORMATION FOR
- Residents & Fellows
- Researchers
Sleep’s Crucial Role in Preserving Memory
Anyone who’s had a poor night’s rest can attest that the lack of sleep impairs cognition, especially memory. But researchers don’t really know why, and unknowns like these complicate the scientific understanding of memory-related conditions like Alzheimer’s disease and other types of dementia.
Most research shows that sleep plays a critical role in the formation and storage of long-term memories. Different types of memories seem to be processed in different brain regions during certain stages of sleep, especially such phases as rapid eye movement (REM) and slow-wave sleep. Furthermore, sleep has another important function: giving the brain a chance to clean itself. Yale researchers take various approaches to understand how sleep shapes our memories.
How Episodic Memories Form and Develop
George Dragoi, MD, PhD , associate professor of psychiatry and of neuroscience at Yale School of Medicine, studies how episodic memories—memories of specific events or experiences—form and develop. Episodic memories complement semantic memories based on facts and general information. They primarily involve parts of the hippocampus and neocortex, and require two separate phases: encoding and consolidation.
Phase 1: Memory Encoding
During encoding, the brain samples stimuli from the outside world and rapidly encodes them within sequences inside networks of neurons in the hippocampus . Dragoi said that when activated, these connected neurons fire one after another, fleshing out the details of the memory. The amygdala seems to attach emotional significance to these memories or details as appropriate at some point along the way.
Phase 2: Memory Consolidation
In consolidation, a process that researchers think occurs during sleep, particularly slow-wave sleep, encoded sequences are integrated by chemical connections into new and existing neuronal knowledge networks and filed for long-term storage in the neocortex. That means that sleep is essential for episodic memory formation, and likely for most types of memory formation. “Encoding is certainly required but not sufficient for [episodic] memory formation,” Dragoi explained. “If encoded information is not consolidated after exposure to new experiences, you simply won’t remember it.”
Sleep’s Critical Role in Memory Consolidation
Why is sleep so essential to consolidation? Possibly because sleep seems to offer optimal conditions for consolidation, providing periods of reduced external stimulation and increased levels of neurotransmitters that promote communication between the hippocampus and the neocortex.
Sleep may also give the brain time to make space for new memories by removing or reducing the strength of neural links tied to memories that are no longer useful. During human development, a process called pruning culls excess neuronal links. “Like in a tree you cut the branches or remove connections in the brain long term,” Dragoi explained. “In adults, the structure of the connections is already built, and the strength of these connections can be reduced or increased over time.”
Based on his work and that of others, Dragoi thinks that sleep may aid this mental tidying-up process, scaling back increased neuronal activity from exposure to specific stimuli and maintaining homeostatic balance in the brain. He adds that some studies also show that the brain seems to produce the templates for proteins according to a kind of internal clock, but that these templates aren’t translated into actual proteins without sleep. “This seems to further link the need for sleep with healthy synaptic function and protein production,” he concluded, a finding that could have wide-ranging therapeutic applications.
Removing the Brain’s Waste Metabolites During Sleep
The question of how memories are lost remains a major focus of memory and sleep research. Using techniques like magnetic resonance imaging (MRI) and electroencephalography (EEG), Helene Benveniste, MD, PhD , professor of anesthesiology, and her colleagues have found that sleep may allow the brain critical time and conditions to remove waste metabolites. The accumulation of certain metabolites in the brain, in particular beta-amyloid and abnormal tau proteins, seems to increase the risk of cognitive disorders like Alzheimer’s disease .
Benveniste said researchers once thought the primary purpose of sleep is to allow rest and memory processing. “Now I think we’re understanding another purpose of sleep may also be to give the brain time to clean itself,” she said.
How Sleep Quality May Affect Alzheimer’s Risk
In 2013, Benveniste helped to initially describe the glymphatic system, a waste-removal pathway in the brain that acts like the lymphatic system but relies largely on astroglial brain cells. In a nutshell, the glymphatic system allows cerebrospinal fluid to flow into the spaces around arteries before passing through aquaporin-4 (AQP4) water channels into brain tissues, where it mixes with fluids and metabolic waste around cells, and then moves out of brain tissues into the space surrounding veins for clearance through the lymphatic or circulatory system.
Benveniste and others have found evidence that healthy glymphatic function may reduce the effects of risk factors implicated in cognitive conditions like Alzheimer’s disease by facilitating metabolic waste clearance in the brain. A study she worked on in 2018 concluded that even one night of sleep deprivation increases beta-amyloid burden in the right hippocampus of adults.
Some studies, including several Benveniste co-authored, also show that many people with Alzheimer’s disease, or cognitive impairment from vascular dementia or small-vessel disease, experience glymphatic dysfunction. The system also seems to be most efficient during sleep, in particular slow-wave sleep, also known as deep sleep. “By far the biggest differences we’ve seen in glymphatic transport rates are when people go from being awake to asleep,” Benveniste explained, adding that medications that manipulate arousal states like anesthetics also seem to heavily influence glymphatic function.
Factors that influence circulation also appear to affect glymphatic function, including pulse rate, breathing rate, and some metabolic conditions. Benveniste and others have also found that glymphatic efficiency improves when rats and other subjects sleep lying on their sides or backs. She noted these positions are the preferred sleeping posture in many animals, including humans.
“This is something I find very interesting clinically,” Benveniste said. “People in the ICU that have trouble swallowing or protecting their airways or have brain trauma are often kept seated with the head raised—even while sleeping. Sometimes we even interrupt sleep frequently to check up on them.” With a better understanding of sleep and glymphatic function, Benveniste explained, practices like these may be revised.
SLEEP-SMART to Improve Sleep Hygiene
As sleep’s effects on memory become clearer, researchers are studying how sleep interventions affect elements of cognition, especially memory difficulties. Hilary Blumberg, MD , the John and Hope Furth Professor of Psychiatric Neuroscience and the director of Yale’s Mood Disorders Research Program, is the principal investigator of a study exploring how behavioral techniques to improve sleep influence cognitive and emotional aging in women . She is collaborating in this research with Carolyn M. Mazure, PhD , the Norma Weinberg Spungen and Joan Lebson Bildner Professor in Women’s Health Research and director of Women’s Health Research at Yale, in a study funded by Women’s Health Access Matters.
Women experience higher rates of sleep disturbances than men , especially as they age—something Blumberg helped link to the fact that women’s frontotemporal brain circuitry seems to be more sensitive to the negative effects of stress than men’s. Frontotemporal brain circuitry involves parts of the amygdala and hippocampus, the brain regions most responsible for cognitive and emotional functioning.
Blumberg said that a focus of her study is teaching people strategies they can use throughout their lives to regularize sleep and other daily activity patterns, which may improve emotional regulation, stress resilience, and cognitive functioning. Focusing on women aged 50 years and over, the study employs a modified version of social rhythm therapy called SLEEP-SMART (Sleep Self-Monitoring And Regulation Therapy) , with sessions that review daily activities and interactions and then modifies them to increase regularity.
This review involves tracking such details as the person someone first comes into contact with each day; mealtimes; and all other daily activities that influence biological rhythms. It also involves using simple self-regulating activities like getting exposure to sunshine before anything else; scheduling important daytime activities; and of course, turning off technological devices before bed.
Study subjects participate in MRI scans before and after the 12-week intervention, which along with other data will identify changes in emotional regulation, cognition, and brain circuitry. These data will allow the team to assess the impact of SLEEP-SMART interventions on cognitive and emotional health, as well as blood and brain biomarkers considered risk factors for Alzheimer’s disease and related dementias.
Investigating Sleep’s Overall Impact on Memory
Researchers have a lot of work to do before they reveal the full intricacies of memory and the extent to which sleep shapes this defining neurological process. Dragoi and his team are following up on their 2019 work, which used electrophysiology to uncover three developmental stages that allow rodent brains to form neuronal sequences used in memory formation. The team hopes to understand what controls shifts between these stages, which could improve the scientific understanding of conditions in which memory is altered, or when internally generated representations like memories dominate the cognitive state, as in autism or schizophrenia .
Blumberg and her team have seen preliminary evidence that regularizing sleep patterns can improve the functioning of the brain circuitry that regulates emotion. She hopes to expand this work, writing that “teaching healthier, more regular sleep and daily activity patterns is something that could be disseminated globally and have a wide impact in improving emotional and cognitive health.”
Benveniste said that researchers will likely need a better understanding of the relationships among glymphatic function, memory, and sleep before therapeutically manipulating these processes. She added that researchers specifically need to know whether slow-wave sleep really boosts waste clearance in the brain. “If this is the case,” she explained, “this is where the focus should be going forward.”
Originally published July 15, 2020; updated May 10, 2022
- Basic Science Research
Featured in this article
- Hilary Blumberg, MD John and Hope Furth Professor of Psychiatric Neuroscience and Professor of Psychiatry, and in the Child Study Center and of Radiology and Biomedical Imaging; Director, Mood Disorders Research Program
- George Dragoi, MD, PhD Associate Professor of Psychiatry and of Neuroscience
- Carolyn M. Mazure, PhD Norma Weinberg Spungen and Joan Lebson Bildner Professor in Women's Health Research and Professor of Psychiatry and of Psychology
Click through the PLOS taxonomy to find articles in your field.
For more information about PLOS Subject Areas, click here .
Loading metrics
Open Access
Peer-reviewed
Research Article
Sleep Improves Memory: The Effect of Sleep on Long Term Memory in Early Adolescence
* E-mail: [email protected] (WEB); [email protected] (KTP)
Affiliation Department of Human Biology, Brown University, Providence, Rhode Island, United States of America
Affiliation Department of Psychiatry and Human Behavior, University of California Irvine, Irvine, California, United States of America
- Katya Trudeau Potkin,
- William E. Bunney Jr
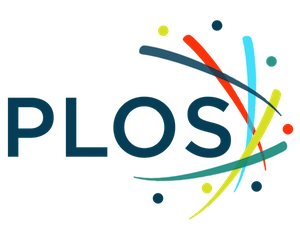
- Published: August 7, 2012
- https://doi.org/10.1371/journal.pone.0042191
- Reader Comments
Sleep plays an important role in the consolidation of memory. This has been most clearly shown in adults for procedural memory (i.e. skills and procedures) and declarative memory (e.g. recall of facts). The effects of sleep and memory are relatively unstudied in adolescents. Declarative memory is important in school performance and consequent social functioning in adolescents. This is the first study to specifically examine the effects of normal sleep on auditory declarative memory in an early adolescent sample. Given that the majority of adolescents do not obtain the recommended amount of sleep, it is critical to study the cognitive effects of normal sleep. Forty male and female normal, healthy adolescents between the ages of ten and fourteen years old were randomly assigned to sleep and no sleep conditions. Subjects were trained on a paired-associate declarative memory task and a control working memory task at 9am, and tested at night (12 hours later) without sleep. The same number of subjects was trained at 9pm and tested 9am following sleep. An increase of 20.6% in declarative memory, as measured by the number correct in a paired-associate test, following sleep was observed compared to the group which was tested at the same time interval without sleep (p<0.03). The performance on the control working memory task that involved encoding and memoranda manipulation was not affected by time of day or relationship to sleep. Declarative memory is significantly improved by sleep in a sample of normal adolescents.
Citation: Potkin KT, Bunney WE Jr (2012) Sleep Improves Memory: The Effect of Sleep on Long Term Memory in Early Adolescence. PLoS ONE 7(8): e42191. https://doi.org/10.1371/journal.pone.0042191
Editor: Antonio Verdejo García, University of Granada, Spain
Received: February 10, 2012; Accepted: July 4, 2012; Published: August 7, 2012
Copyright: © Potkin, Bunney Jr. This is an open-access article distributed under the terms of the Creative Commons Attribution License, which permits unrestricted use, distribution, and reproduction in any medium, provided the original author and source are credited.
Funding: The authors have no support or funding to report.
Competing interests: The authors have declared that no competing interests exist.
Introduction
Several studies primarily in adults have shown that sleep improves procedural memory, i.e. skills and procedures [1] , [2] as well as declarative memory [3] . REM and slow-wave sleep (SWS) have been implicated in memory consolidation [3] – [5] . Lack of REM sleep is associated with poor recall of visual location [6] . Decline in declarative memory consolidation is correlated with a decline in slow-wave sleep [7] . Spencer et al. observed similar initial procedural learning in older and younger adults; however, the older adults’ performance did not improve following sleep, suggesting that sleep dependent memory consolidation decreases with age [8] . This may reflect the disturbed sleep and disrupted SWS in the elderly [3] , [8] , [9] . Slow wave sleep increases until shortly before puberty and then shows a prominent drop across adolescence, decreasing by more than 60% between ages 10 and 20 years [10] . It is critical to understand the cognitive effects of normal sleep in order to understand the consequences of disrupted sleep. This is important since the majority of adolescents do not obtain the recommended amount of sleep and that disrupted sleep is a key symptom in most adolescent psychiatric and developmental disorders [11] .
Backhaus et al. studied twenty-seven children with an average age of 10.1 years (range of nine to twelve), on a learned word pairs list, employing a within subject design and two post-learning assessments. They found that declarative memory was significantly increased immediately after an interval of sleep, as well as with delayed post-learning sleep [12] . As the authors had noted, no control task was administered to determine if circadian confounds were responsible for this increase in recall post sleep. Our study addressed this limitation by administering a control task and evaluating the effect of sleep on auditory declarative memory consolidation in early adolescence. Visual declarative memory has been reported to be enhanced following sleep in children; however, auditory declarative memory has not been previously studied [13] .
Participants
Twenty female and twenty male adolescents, between the ages of 10 and 14, were recruited in a public middle school. The study was considered exempt by the institutional review board because it involved the use of educational tests without personal subject identifiers. In accord with the principals of the Declaration of Helsinki, subjects were asked to participate in a school class project and only told that they would be tested two times for about 15 minutes each time. Subjects with academic failure or accelerated academic performance or sleep problems were not included. The subjects agreeing to participate were grouped by sex and assigned to sleep or no sleep conditions with a separate randomization table for each group, to ensure a balanced design.
Subjects were tested in their homes in a quiet room without distractions for the duration of the learning and testing. The testing sessions were conducted during weekends or during school break. All subjects were given the paired-associate test, one of the standard tests of declarative memory [14] , which consisted of repeating semantically related and unrelated pairs of words (e.g. tree/leaf; lamp/shoe), in a standardized manner. After each word pair was presented out loud, the subject repeated the pair out loud to ensure registration of the paired associate. The list of the same 10 pairs was administered three times in immediate succession. Subjects assigned to the sleep condition learned the paired associates at 9∶00pm (±30 minutes), and were tested for cued recall twelve hours later, after a night of sleep. The no-sleep group received the same paired-associate presentation at 9∶00am (±30 minutes) and was tested for recall twelve hours later, with no intervening sleep or naps. The control working memory task, letter-number, was given just prior to learning the paired-associate words and again just prior to being tested on the paired-associate words. The letter-number test was administered to control for possible circadian confounds and to control for attention and encoding. The letter-number control task (LN, immediate recall and reordering of letters and numbers) is a subtest of the WAIS-III (Wechsler Adult Intelligence Scale) and WMS-III (Wechsler memory Scale), the most widely used intelligence and memory scales. An increasing long series of mixed letters and numbers is read to the subject and the subject then orders the numbers and letters in ascending order, e.g. b3a1 is read and subject correctly responds with 13ab. The letters and numbers must be encoded and then manipulated to get the correct answer. Two versions of the letter-number task were used in random order. The number correct was scored for the paired-associate and the letter-number tests. The memory scores were transformed into Z scores to determine if outliers were present; an exclusionary Z score of ±2.57 was applied (1% of the normal distribution). Between group comparisons were calculated by students t-test (2 tailed) after testing for equal variances by Levene’s test, and ANCOVA as necessary. Within subject comparisons were calculated by paired t-test.
Subjects were instructed to eat their usual meals approximately one hour before learning the paired-associates and one hour before being tested on the paired-associates. Subjects were instructed to get a good night’s sleep. All the subjects included reported having had typical night of sleep and rated the quality of the sleep as good to very good prior to the testing.
The sleep group’s mean age was 12.9 compared to 12.4 for the non-sleep group (t = (1.52), df (1,38), p = 0.14). (See Table 1 for demographic characteristics and performance scores). There was no statistically significant sex difference in performance for either task.
- PPT PowerPoint slide
- PNG larger image
- TIFF original image
https://doi.org/10.1371/journal.pone.0042191.t001
Three outliers were identified and removed; one high scoring subject assigned to the sleep and two lower scoring subjects assigned to no sleep. After removing outliers, 19 sleep subjects and 18 no- sleep subjects remained. The Levene’s Test showed equality of variances for all comparisons. The number correct on the letter-number control task at initial testing was 6.58 for the sleep group and 6.06 for the no-sleep group, (t = (1.54), df (1,35), p = 0.13). The letter-number correct score on the second administration was 6.26 and 6.33, respectively, (t = (-.16), df (1,35), p = 0.88), ( Figure 1 ). There was also no statistically significant difference in performance for either group on letter-number task between the first and second administration (paired t test, p = 0.32 for sleep group and 0.45 for no-sleep group).
A histogram of mean number correct (± SD) for the Paired-Associate Test (PA) and Letter Number Test (Letter #), with (n = 19) (outliers removed) and without sleep (n = 18).
https://doi.org/10.1371/journal.pone.0042191.g001
An increase of 20.6% in long-term memory ( Figure 1 ) was found as measured by the number correct in the paired-associate test following sleep, compared to the group which was tested at the same time interval, but without sleep (p<0.029). When the three outliers are included, the number correct for recall of the paired-associates was statistically greater for the sleep group (7.5) compared to the no sleep group (5.9, t = (2.76), df (1,37), p<0.009), a 32.7% increase.
The paired-associate test is one of the standard tests of declarative memory and has been previously used to study declarative memory and the effects of sleep on declarative memory in adults and children [3] . All subjects were evaluated at the same two times of day, approximately 9 AM and 9 PM, using standardized conditions. Performance on the paired-associate test was significantly affected by sleep in our adolescent sample. In contrast, working memory performance as measured by the letter-number test, a standard subtest of the WAIS-III and WMS-III was not affected by time of day or in relation to sleep. Correct performance on the letter-number working memory task (LN) requires that the letters and numbers presented to the subject must be encoded and then correctly manipulated. We had 80% power to detect a standardized difference of.76 correct (∼11% change) or greater, following sleep. A small difference in working memory performance (<11%), however, may exist that could not be detected with our sample size.
The equal performance at both sessions and between groups on the LN supports the view that equal registration and encoding of the memoranda was comparable at both time points and between groups. Performance on the working memory control task did not change with the second session for either group, suggesting that the time of day had no effect on performance on the working memory control task. Consequently, the observed difference in paired-associate performance, i.e. consolidation of working memory, is most likely related to sleep itself and not any differences in encoding. Memory consolidation has been reported to be affected by sleep [1] , [2] , [8] , [9] . Both REM and slow-wave sleep have been associated with improved memory [3] – [5] . Slow wave sleep particularly enhances declarative memory. 7 .
Our results are consistent with Gais et al.’s study of young males (mean age 17.4) showing that enhanced declarative memory was related to periods of sleep, and not to time of day effects [15] . Naps improve declarative memory regardless of time of nap [16] and closely resembled memory improvement after an eight-hour night of sleep [17] . In reviewing the timing of sleep and circadian rhythms, Diekelmann et al. conclude that sleep promotes memory consolidation independently of the time of day in which it occurs [3] . Voderholzer et al. studying 14–16 year old adolescents showed that several nights of sleep restriction did not impact memory consolidation nor performance in a working memory task, when two recovery night of sleep were provided, an effect they ascribed to a compensatory enhancement of SWS [18] . The paired-associate test begins as a working memory task and after a period of time with consolidation becomes a declarative memory task. Correct performance on the letter-number test and the paired-associate tests are dependent upon encoding the memoranda.
A limitation of this study is that we did not test for encoding strength by immediate recall after the administration of the paired-associate test. The letter-number test requires attention and encoding. An element of immediate recall is to prove that the subject was attending. This was assured by having the subjects read the words (similar to other learning tests like the CERAD and ADAS-COG) and supported by the finding of the performance on the letter-number test. It is likely that if immediate recall following each presentation was obtained, higher accuracy rates would have been observed. Recent studies have demonstrated that salience increases declarative memory performance [13] , [19] . Nevertheless, our data demonstrate that sleep improves memory consolidation even in conditions where encoding has not been reinforced.
Neither time of day or sleep affected the performance on the letter-number test suggesting that the material was being learned and encoded. There is no evidence that memory consolidation depends on time of day independent of sleep. The lack of interference during sleep has been considered as a possible cause of the beneficial effects of sleep on declarative memory, i.e. there are no daytime demands to interfere with memory consolidation. Our design tested subjects on non-school days, thus mitigating the effects of interference of memory consolidation during the day by learning competition and other demands of a normal school day. Gais et al. controlled for waking associated interference and found no effect of interference on memory [15] . In a review of controversy regarding whether absence of interference accounts for memory improvement during sleep, Ellenbogen at al. point out “although sleep might passively protect declarative memories from interference, consolidation must also occur during sleep for the memories to become resistant to interference the following day”. Based on their review of related animal and human studies, they point out that “hippocampus-dependent memories are reactivated during sleep, and that this reactivation leads to strengthened memory traces”, finally concluding “that specific, sleep-dependent, neurobiological processes directly lead to the consolidation of declarative memories” [1] . Diekelmann et al. hypothesized that both encoding and sleep-dependent consolidation during sleep involve prefrontal-hippocampal circuitry [3] .
Children have high amounts of slow wave sleep and sleep in general. Sleep has been shown to improve declarative and procedural memory in children and older age groups. Subjects were asked about their sleep and confirmed that they had a typical night sleep, consisting of 8–10 hours of sleep, average for adolescents [20] . We did not, however, specifically measure sleep. Lack of sleep can result in poor cognitive performance, which was not observed in our sample, and is consistent with the subjects’ report of a good night sleep and that poor sleepers were excluded from the sample.
A cross-over design would have provided additional confirmation at the individual subject level in contrast to our parallel group design. Our study was limited as the sample was opportune, from a California middle school, and was not epidemiologically based. No subjects approached declined to participate. No accelerated or failing students were included, although this was not a strict exclusion criterion. There were 3% African-American, 5% Asian, and 92% Caucasian. The sample population reflected the general school population in this geographic area, although Asians were underrepresented (12.8%).
Our sample size was relatively small and limited to early adolescence, ages 10–14, although twice the sample of Prehn-Kristensen et al. who found 10 to 13 year olds improved visual memory following sleep, especially to emotional pictures [13] . The 10–14 age group was deliberately chosen because of the importance of declarative memory on adolescent school performance and related social functioning [21] . Marked changes in sleep and sleep architecture are a defining feature of adolescence [22] . Disorders of adolescence frequently disrupt sleep. Twenty-five to forty percent of adolescents have sleep disorders that can have an important effect on daytime school and consequent social functioning [23] . Sleep disorders are even more prevalent in adolescents with psychiatric disorders and developmental disabilities [24] . It is important to have data on the effects of normal sleep on declarative memory in normal adolescents to better understand the consequences of lack of sleep and abnormal sleep patterns.
Given the importance of adolescent memory on school performance and consequent social functioning, a fuller understanding of the effects of sleep on memory consolidation is needed. Other studies are needed to investigate the specific effects of sleep on other types of memory, such as visual, procedural, and emotional. Understanding the role of normal sleep on memory consolidation in adolescence is critical in identifying the consequences of disrupted sleep in adolescent disorders and their treatment.
Author Contributions
Conceived and designed the experiments: KTP WEB. Performed the experiments: KTP. Analyzed the data: KTP WEB. Contributed reagents/materials/analysis tools: KTP WEB. Wrote the paper: KTP WEB.
- View Article
- Google Scholar
How the brain chooses which memories are important enough to save and which to let fade away
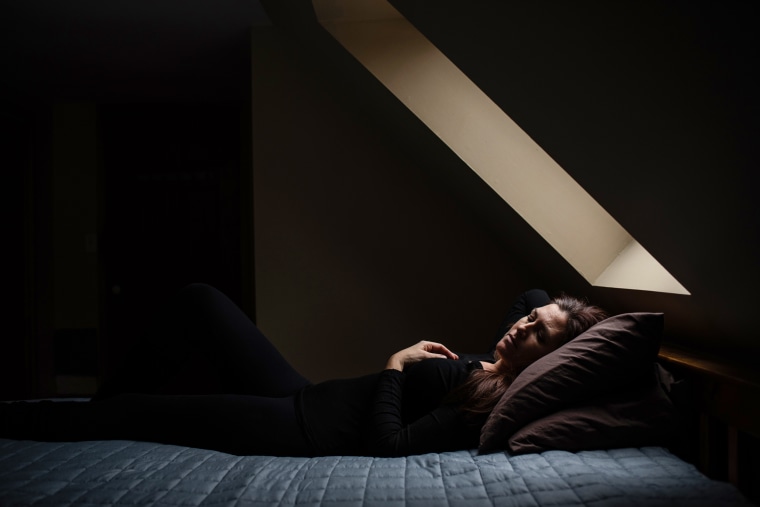
Memory can be mysterious. Certain life events remain clear in our minds no matter how long ago they occurred, while episodes from the prior day may already be fuzzy and difficult to recall.
A study, published Thursday in Science, reveals why this happens. Scientists have found that the brains of humans and other mammals have a system for choosing which life experiences are important enough to be cemented into long-term memory — and which will be allowed to fade away.
Experiments in mice revealed that during waking hours, cells in the brain’s hippocampus spark in a specific pattern called "sharp-wave ripples," which tag important experiences for movement into long-term memory storage during sleep.
Although the research was performed in mice, certain brain processes have remained almost the same as mammals have evolved, so the findings can tell us a lot about ourselves, said the study’s senior author, Dr. György Buzsáki , Biggs Professor of Neuroscience at NYU Langone Health.
As part of the research, Buzsáki and his colleagues put mice through a maze that had a sugary reward at the end for those that successfully reached it. Meanwhile, the researchers were monitoring the activity of nerve cells through electrodes implanted in the rodent brains that fed data into computer programs.
They observed that as the mice paused to eat their treats, their brains sparked sharp-wave ripples that were repeated as many as 20 times. The daytime pattern of sharp-wave ripples was replayed during the night, a process that moved the experience into long-term memory.
It's during sleep when experiences from waking hours deemed to be important are converted into enduring memories.
Events that were followed by very few or no sharp-wave ripples failed to form lasting memories, the researchers noted.
This tagging process during waking hours is totally unconscious, Buzsáki said. “The brain decides on its own, rather than us deciding voluntarily,” he added.
Relaxation needed for long-term memory
Still, the research suggests there are things we can do to increase the likelihood of a memory being stored permanently.
If, like the mice, we pause after an experience, it may help cement the events into long-term memory, Buzsáki said. We need that period of relaxation , when we’re not paying close attention to anything, to allow sharp-wave ripples to spark. That wakeful process is an essential part of creating a permanent memory .
Practically speaking, this means if you like to binge TV series, you’re not likely to remember much of any episode except the last one you watched, Buzsáki said.
“If you watch a movie and would like to remember it, it’s better to go for a walk afterwards,” Buzsáki said. “No double features.”
An intriguing finding of the new research is the discovery that there could be bursts of activity — the sharp-wave ripples — when the mouse is standing still and its brain is essentially idling, said Daniela Schiller, a professor of neuroscience and psychiatry at the Icahn School of Medicine at Mount Sinai.
What’s amazing, Schiller said, is the pattern recorded close to the event was the same as what was seen during sleep. And both patterns mimicked the mouse’s real-life experience.
The study showed that events followed by a pause and electrical bursts in the brain are the ones that we will find in long-term memory, said Daphna Shohamy, director of Columbia University’s Zuckerman Institute. If you observe animals, you can see them pausing during the day after a novel or rewarding experience, she said.
“We did a study a few years ago in which we had humans navigate a maze with random objects along the way, looking for a treasure,” Shohamy said. “If they got the treasure, they were more likely to remember the random object they passed along the way.”
Linda Carroll is a regular health contributor to NBC News. She is coauthor of "The Concussion Crisis: Anatomy of a Silent Epidemic" and "Out of the Clouds: The Unlikely Horseman and the Unwanted Colt Who Conquered the Sport of Kings."
Thank you for visiting nature.com. You are using a browser version with limited support for CSS. To obtain the best experience, we recommend you use a more up to date browser (or turn off compatibility mode in Internet Explorer). In the meantime, to ensure continued support, we are displaying the site without styles and JavaScript.
- View all journals
- Explore content
- About the journal
- Publish with us
- Sign up for alerts
- Published: 07 July 2022
Memory-enhancing properties of sleep depend on the oscillatory amplitude of norepinephrine
- Celia Kjaerby ORCID: orcid.org/0000-0003-1106-9217 1 na1 ,
- Mie Andersen ORCID: orcid.org/0000-0002-6978-585X 1 na1 ,
- Natalie Hauglund ORCID: orcid.org/0000-0002-2198-6329 1 ,
- Verena Untiet ORCID: orcid.org/0000-0002-1888-6378 1 ,
- Camilla Dall 1 ,
- Björn Sigurdsson ORCID: orcid.org/0000-0002-7484-7779 1 ,
- Fengfei Ding 2 , 3 ,
- Jiesi Feng 4 ,
- Yulong Li ORCID: orcid.org/0000-0002-9166-9919 4 , 5 , 6 ,
- Pia Weikop 1 ,
- Hajime Hirase ORCID: orcid.org/0000-0003-3806-6905 1 &
- Maiken Nedergaard ORCID: orcid.org/0000-0001-6502-6031 1 , 2
Nature Neuroscience volume 25 , pages 1059–1070 ( 2022 ) Cite this article
18k Accesses
65 Citations
412 Altmetric
Metrics details
- Consolidation
- Locus coeruleus
- Non-REM sleep
- Wakefulness
Matters Arising to this article was published on 20 April 2023
Sleep has a complex micro-architecture, encompassing micro-arousals, sleep spindles and transitions between sleep stages. Fragmented sleep impairs memory consolidation, whereas spindle-rich and delta-rich non-rapid eye movement (NREM) sleep and rapid eye movement (REM) sleep promote it. However, the relationship between micro-arousals and memory-promoting aspects of sleep remains unclear. In this study, we used fiber photometry in mice to examine how release of the arousal mediator norepinephrine (NE) shapes sleep micro-architecture. Here we show that micro-arousals are generated in a periodic pattern during NREM sleep, riding on the peak of locus-coeruleus-generated infraslow oscillations of extracellular NE, whereas descending phases of NE oscillations drive spindles. The amplitude of NE oscillations is crucial for shaping sleep micro-architecture related to memory performance: prolonged descent of NE promotes spindle-enriched intermediate state and REM sleep but also associates with awakenings, whereas shorter NE descents uphold NREM sleep and micro-arousals. Thus, the NE oscillatory amplitude may be a target for improving sleep in sleep disorders.
This is a preview of subscription content, access via your institution
Access options
Access Nature and 54 other Nature Portfolio journals
Get Nature+, our best-value online-access subscription
24,99 € / 30 days
cancel any time
Subscribe to this journal
Receive 12 print issues and online access
195,33 € per year
only 16,28 € per issue
Buy this article
Purchase on Springer Link
Instant access to full article PDF
Prices may be subject to local taxes which are calculated during checkout
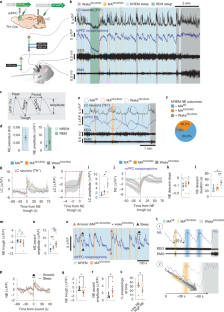
Similar content being viewed by others
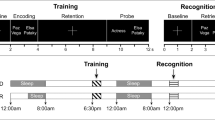
Weakly encoded memories due to acute sleep restriction can be rescued after one night of recovery sleep
Daniel Baena, Jose L. Cantero, … Mercedes Atienza
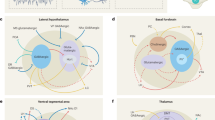
Neuro-orchestration of sleep and wakefulness
Bibi A. Sulaman, Su Wang, … Ada Eban-Rothschild
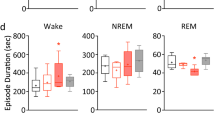
Chronic sleep fragmentation enhances habenula cholinergic neural activity
Feifei Ge, Ping Mu, … Yanhua H. Huang
Data availability
The datasets generated during and/or analyzed in this study are available from the corresponding author upon reasonable request. Source data are provided with this paper.
Code availability
The custom-made MATLAB code used in this study is available from GitHub: https://github.com/MieAndersen/NE-oscillations .
Andrillon, T. et al. Sleep spindles in humans: insights from intracranial EEG and unit recordings. J. Neurosci. 31 , 17821–17834 (2011).
Article CAS PubMed PubMed Central Google Scholar
Fernandez, L. M. J. & Lüthi, A. Sleep spindles: mechanisms and functions. Physiol. Rev. 100 , 805–868 (2020).
Bonnet, M. H. & Arand, D. L. EEG arousal norms by age. J. Clin. Sleep. Med. 3 , 271–274 (2007).
Article PubMed PubMed Central Google Scholar
Boselli, M., Parrino, L., Smerieri, A. & Terzano, M. G. Effect of age on EEG arousals in normal sleep. Sleep 21 , 351–357 (1998).
CAS PubMed Google Scholar
Bonnet, M. H. Effect of sleep disruption on sleep, performance, and mood. Sleep 8 , 11–19 (1985).
Article CAS PubMed Google Scholar
Rauchs, G. et al. Is there a link between sleep changes and memory in Alzheimer’s disease? Neuroreport 19 , 1159–1162 (2008).
Landolt, H. P., Dijk, D. J., Achermann, P. & Borbély, A. A. Effect of age on the sleep EEG: slow-wave activity and spindle frequency activity in young and middle-aged men. Brain Res. 738 , 205–212 (1996).
Soltani, S. et al. Sleep–wake cycle in young and older mice. Front. Syst. Neurosci. 13 , 51 (2019).
Saper, C. B., Fuller, P. M., Pedersen, N. P., Lu, J. & Scammell, T. E. Sleep state switching. Neuron 68 , 1023–1042 (2010).
Constantinople, C. M. & Bruno, R. M. Effects and mechanisms of wakefulness on local cortical networks. Neuron 69 , 1061–1068 (2011).
Aston-Jones, G. & Bloom, F. E. Activity of norepinephrine-containing locus coeruleus neurons in behaving rats anticipates fluctuations in the sleep–waking cycle. J. Neurosci. 1 , 876–886 (1981).
Hobson, J. A., McCarley, R. W. & Wyzinski, P. W. Sleep cycle oscillation: reciprocal discharge by two brainstem neuronal groups. Science 189 , 55–58 (1975).
Eschenko, O. & Sara, S. J. Learning-dependent, transient increase of activity in noradrenergic neurons of locus coeruleus during slow wave sleep in the rat: brain stem–cortex interplay for memory consolidation? Cereb. Cortex 18 , 2596–2603 (2008).
Article PubMed Google Scholar
Eschenko, O., Magri, C., Panzeri, S. & Sara, S. J. Noradrenergic neurons of the locus coeruleus are phase locked to cortical up–down states during sleep. Cereb. Cortex 22 , 426–435 (2012).
Carter, M. E. et al. Tuning arousal with optogenetic modulation of locus coeruleus neurons. Nat. Neurosci. 13 , 1526–1533 (2010).
Rasmussen, K., Morilak, D. A. & Jacobs, B. L. Single unit activity of locus coeruleus neurons in the freely moving cat. I. During naturalistic behaviors and in response to simple and complex stimuli. Brain Res. 371 , 324–334 (1986).
Foote, S. L., Aston-Jones, G. & Bloom, F. E. Impulse activity of locus coeruleus neurons in awake rats and monkeys is a function of sensory stimulation and arousal. Proc. Natl Acad. Sci. USA 77 , 3033–3037 (1980).
Shouse, M. N., Staba, R. J., Saquib, S. F. & Farber, P. R. Monoamines and sleep: microdialysis findings in pons and amygdala. Brain Res. 860 , 181–189 (2000).
Léna, I. et al. Variations in extracellular levels of dopamine, noradrenaline, glutamate, and aspartate across the sleep–wake cycle in the medial prefrontal cortex and nucleus accumbens of freely moving rats. J. Neurosci. Res. 81 , 891–899 (2005).
Yamaguchi, H., Hopf, F. W., Li, S.-B. & Lecea, Lde In vivo cell type-specific CRISPR knockdown of dopamine beta hydroxylase reduces locus coeruleus evoked wakefulness. Nat. Commun. 9 , 5211 (2018).
Rajkowski, J., Kubiak, P. & Aston-Jones, G. Locus coeruleus activity in monkey: phasic and tonic changes are associated with altered vigilance. Brain Res. Bull. 35 , 607–616 (1994).
Swift, K. M. et al. Abnormal locus coeruleus sleep activity alters sleep signatures of memory consolidation and impairs place cell stability and spatial memory. Curr. Biol. 28 , 3599–3609 (2018).
Lecci, S. et al. Coordinated infraslow neural and cardiac oscillations mark fragility and offline periods in mammalian sleep. Sci. Adv. 3 , e1602026 (2017).
Osorio-Forero, A. et al. Noradrenergic circuit control of non-REM sleep substates. Curr. Biol. 31 , 5009–5023 (2021).
Novitskaya, Y., Sara, S. J., Logothetis, N. K. & Eschenko, O. Ripple-triggered stimulation of the locus coeruleus during post-learning sleep disrupts ripple/spindle coupling and impairs memory consolidation. Learn. Mem. 23 , 238–248 (2016).
Feng, J. et al. A genetically encoded fluorescent sensor for rapid and specific in vivo detection of norepinephrine. Neuron 102 , 745–761 (2019).
Osorio-Forero, A. et al. Infraslow locus coeruleus activity coordinates spindle rhythms and heart rate to gate fluctuating non-REM sleep substates. Preprint at https://www.biorxiv.org/content/10.1101/2021.03.08.434399v1 (2021).
EEG arousals: scoring rules and examples: a preliminary report from the sleep disorders atlas task force of the american sleep disorders association. Sleep 15 , 173–184 (1992).
Gottesmann, C. The transition from slow-wave sleep to paradoxical sleep: evolving facts and concepts of the neurophysiological processes underlying the intermediate stage of sleep. Neurosci. Biobehav. Rev. 20 , 367–387 (1996).
Krueger, J. N. et al. Amnesia for context fear is caused by widespread disruption of hippocampal activity. Neurobiol. Learn. Mem. 175 , 107295 (2020).
Takeuchi, T. et al. Locus coeruleus and dopaminergic consolidation of everyday memory. Nature 537 , 357–362 (2016).
Mahn, M., Prigge, M., Ron, S., Levy, R. & Yizhar, O. Biophysical constraints of optogenetic inhibition at presynaptic terminals. Nat. Neurosci. 19 , 554 (2016).
de Medeiros, M. A., Reis, L. C. & Mello, L. E. Stress-induced c-Fos expression is differentially modulated by dexamethasone, diazepam and imipramine. Neuropsychopharmacology 30 , 1246–1256 (2005).
Mayers, A. G. & Baldwin, D. S. Antidepressants and their effect on sleep. Hum. Psychopharmacol. 20 , 533–559 (2005).
Mitchell, H. A. & Weinshenker, D. Good night and good luck: norepinephrine in sleep pharmacology. Biochem. Pharmacol. 79 , 801–809 (2010).
Watts, A., Gritton, H. J., Sweigart, J. & Poe, G. R. Antidepressant suppression of non-REM sleep spindles and REM sleep impairs hippocampus-dependent learning while augmenting striatum-dependent learning. J. Neurosci. 32 , 13411–13420 (2012).
Porter-Stransky, K. A. et al. Noradrenergic transmission at alpha1-adrenergic receptors in the ventral periaqueductal gray modulates arousal. Biol. Psychiatry 85 , 237–247 (2019).
Rudzik, F. et al. Sleep spindle characteristics and arousability from nighttime transportation noise exposure in healthy young and older individuals. Sleep 41 , zsy077 (2018).
Peter-Derex, L., Yammine, P., Bastuji, H. & Croisile, B. Sleep and Alzheimer’s disease. Sleep. Med. Rev. 19 , 29–38 (2015).
Scammell, T. E., Arrigoni, E. & Lipton, J. O. Neural circuitry of wakefulness and sleep. Neuron 93 , 747 (2017).
Luppi, P. H., Aston-Jones, G., Akaoka, H., Chouvet, G. & Jouvet, M. Afferent projections to the rat locus coeruleus demonstrated by retrograde and anterograde tracing with cholera-toxin B subunit and Phaseolus vulgaris leucoagglutinin. Neuroscience 65 , 119–160 (1995).
Aston-Jones, G., Ennis, M., Pieribone, V. A., Nickell, W. T. & Shipley, M. T. The brain nucleus locus coeruleus: restricted afferent control of a broad efferent network. Science 234 , 734–737 (1986).
Picchioni, D. et al. Autonomic arousals contribute to brain fluid pulsations during sleep. Neuroimage 249 , 118888 (2022).
Fultz, N. E. et al. Coupled electrophysiological, hemodynamic, and cerebrospinal fluid oscillations in human sleep. Science 366 , 628–631 (2019).
Purcell, S. M. et al. Characterizing sleep spindles in 11,630 individuals from the National Sleep Research Resource. Nat. Commun. 8 , 15930 (2017).
Dringenberg, H. C. & Olmstead, M. C. Integrated contributions of basal forebrain and thalamus to neocortical activation elicited by pedunculopontine tegmental stimulation in urethane-anesthetized rats. Neuroscience 119 , 839–853 (2003).
Gervasoni, D. et al. Effect of chronic treatment with milnacipran on sleep architecture in rats compared with paroxetine and imipramine. Pharmacol. Biochem. Behav. 73 , 557–563 (2002).
Jones, B. E. The role of noradrenergic locus coeruleus neurons and neighboring cholinergic neurons of the pontomesencephalic tegmentum in sleep–wake states. Prog. Brain Res. 88 , 533–543 (1991).
Williams, J. A. & Reiner, P. B. Noradrenaline hyperpolarizes identified rat mesopontine cholinergic neurons in vitro. J. Neurosci. 13 , 3878–3883 (1993).
Latchoumane, C.-F. V., Ngo, H.-V. V., Born, J. & Shin, H.-S. Thalamic spindles promote memory formation during sleep through triple phase-locking of cortical, thalamic, and hippocampal rhythms. Neuron 95 , 424–435 (2017).
Li, W., Ma, L., Yang, G. & Gan, W.-B. REM sleep selectively prunes and maintains new synapses in development and learning. Nat. Neurosci. 20 , 427–437 (2017).
Ribeiro, S. et al. Novel experience induces persistent sleep-dependent plasticity in the cortex but not in the hippocampus. Front. Neurosci. 1 , 43–55 (2007).
Giuditta, A. et al. The sequential hypothesis of the function of sleep. Behav. Brain Res. 69 , 157–166 (1995).
Gais, S., Rasch, B., Dahmen, J. C., Sara, S. & Born, J. The memory function of noradrenergic activity in non-REM sleep. J. Cogn. Neurosci. 23 , 2582–2592 (2011).
Rasch, B., Pommer, J., Diekelmann, S. & Born, J. Pharmacological REM sleep suppression paradoxically improves rather than impairs skill memory. Nat. Neurosci. 12 , 396–397 (2008).
Aghajanian, G. K. & VanderMaelen, C. P. α 2 -adrenoceptor-mediated hyperpolarization of locus coeruleus neurons: intracellular studies in vivo. Science 215 , 1394–1396 (1982).
Washburn, M. & Moises, H. C. Electrophysiological correlates of presynaptic alpha 2-receptor-mediated inhibition of norepinephrine release at locus coeruleus synapses in dentate gyrus. J. Neurosci. 9 , 2131–2140 (1989).
Hayat, H. et al. Locus coeruleus norepinephrine activity mediates sensory-evoked awakenings from sleep. Sci. Adv. 6 , eaaz4232 (2020).
Oe, Y. et al. Distinct temporal integration of noradrenaline signaling by astrocytic second messengers during vigilance. Nat. Commun. 11 , 471 (2020).
Download references
Acknowledgements
Funding: Lundbeck Foundation, R386–2021–165; Independent Research Council Denmark, 7016–00324A; Augustinus Foundation, 16–3735.; Novo Nordisk Foundation, NNF20OC0066419; US Department of Health & Human Services, National Institutes of Health (NIH), R01AT011439; US Department of Defense, Army Research Office, W911NF1910280; The Simons Foundation, 811237; Adelson Foundation; National Key R&D Program of China, 2021YFF0502904; National Natural Science Foundation of China, 31925017 and 31871087; NIH BRAIN Initiative, 1U01NS113358 and 1U01NS120824; and the FENG Foundation. We thank Myles Billard for technical support and Dan Xue for graphical assistance.
Author information
These authors contributed equally: Celia Kjaerby, Mie Andersen.
Authors and Affiliations
Division of Glial Disease and Therapeutics, Center for Translational Neuromedicine, University of Copenhagen, Copenhagen, Denmark
Celia Kjaerby, Mie Andersen, Natalie Hauglund, Verena Untiet, Camilla Dall, Björn Sigurdsson, Pia Weikop, Hajime Hirase & Maiken Nedergaard
Division of Glial Disease and Therapeutics, Center for Translational Neuromedicine, Department of Neurosurgery, University of Rochester Medical Center, Rochester, NY, USA
Fengfei Ding & Maiken Nedergaard
Department of Pharmacology, Shanghai Medical College, Fudan University, Shanghai, China
Fengfei Ding
State Key Laboratory of Membrane Biology, Peking University School of Life Sciences, Beijing, China
Jiesi Feng & Yulong Li
PKU-IDG/McGovern Institute for Brain Research, Beijing, China
Peking-Tsinghua Center for Life Sciences, Academy for Advanced Interdisciplinary Studies, Peking University, Beijing, China
You can also search for this author in PubMed Google Scholar
Contributions
Conceptualization: C.K., M.A., H.H., M.N. and P.W. Fiber photometry and EEG methodology and investigation: C.K., M.A., N.H., C.D. and V.U. Viral constructs: Y.L., J.F. and F.D. HPLC methodology: P.W. Analysis: C.K., M.A. and B.S. Visualization: C.K. and M.A. Supervision: H.H. and M.N. Writing—original draft: C.K. and M.A. Writing—review and editing: C.K., M.A., H.H. and M.N.
Corresponding authors
Correspondence to Celia Kjaerby or Maiken Nedergaard .
Ethics declarations
Competing interests.
The authors declare no competing interests.
Peer review
Peer review information.
Nature Neuroscience thanks the anonymous reviewers for their contribution to the peer review of this work.
Additional information
Publisher’s note Springer Nature remains neutral with regard to jurisdictional claims in published maps and institutional affiliations.
Extended data
Extended data fig. 1 all ascending stages of ne oscillations are associated with eeg-defined micro-arousals or awakenings..
a , Mean power traces for delta, theta, sigma and beta frequency bands aligned to NE rise associated with EEG/EMG-based transitions from NREM sleep to continued NREM sleep (blue, MA NE ), micro-arousals (MA EEG/EMG , orange) and wake (grey, wake EEG/EMG ). b , Summary plot showing the reduction in band power across the different transitions. There was no difference between MA NE and MA EEG/EMG for theta, sigma and beta band power, which are the frequencies used to assess micro-arousals. Significance was calculated by means of two-way repeated measures ANOVA with Šídák’s post hoc test (only MA NE and MA EEG/EMG post hoc comparisons shown in graph for simplicity, P = 0.0003, delta; P = 0.13, theta; P = 0.11, sigma; P = 0.65, beta). c , Slope of linear regression on 5 initial seconds of NE rise was used as estimate for rise time. d , NE slope across the different type of transitions (repeated measures one-way ANOVA with Tukey’s multiple comparisons test, P = 0.0059, MA NE vs wake; P = 0.041, MA EEG/EMG vs wake). e , Multi-taper power spectral analysis showed increased power for slower frequencies for NREM sleep compared to wake and REM sleep with no defined peak frequency likely due to the discrete nature of NE oscillations. n = 7. Data is shown as mean ± SEM. * P < 0.05, ** P < 0.01, *** P < 0.001.
Source data
Extended data fig. 2 decrease in sigma power marks tone-evoked arousal..
Based on the local decline in sigma power in response to tone (> 0.1 log(μV 2 )), the tone outcome was divided into arousal and otherwise maintained sleep. a , Mean sigma power traces in response to tone leading to either arousal or persistent sleep. b , The maximum value of sigma power maximum prior to tone (‘pre’) and the minimum sigma power after the tone (‘post’) for the defined arousal and sleep conditions. c , The difference in sigma power occurring in response to the tone. Significance was calculated by means of two-way repeated measures ANOVA with Šídák’s post hoc test ( b , P = 0.035, pre; P = 0.023, post) or two-tailed paired t -test ( c , P = 0.0034). n = 6. Data is shown as mean ± SEM. * P < 0.05, ** P < 0.01.
Extended Data Fig. 3 Relationship between norepinephrine, sigma power, spindles and delta power.
a , Representative trace showing sigma power of surface EEG recordings with corresponding detection of spindles based on S1 cortical LFP recordings. b , Correlation between spindle occurrences and mean sigma power across 10 s bins over a 5 min NREM sleep episode. c , Mean Pearson r values (0.63 ± 0.02). d , Mean sigma power traces normalized to baseline showing the amount of sigma power increase associated with NE descents preceeding microarousals (MA NE or MA EEG/EMG ) or awakenings (wake EEG/EMG ). Calculated area under the curve (AUC) for sigma power is largest during the NE descents associated with awakenings transitions (two-tailed paired t -test; P = 0.60, MA NE vs MA EEG/EMG ; P = 0.0057, MA NE vs wake; P = 0.044, MA EEG/EMG vs wake). e , Mean correlation coefficient between delta power and NE level (5 min NREM sleep episodes). f , Three different example traces showing how optogenetic activation of locus coeruleus (LC, 2 s 20 Hz 10 ms pulses) during periods of NE descend leads to NE ascend followed by a delayed change in spindle occurrences and amplitude reduction of 7–15 bandpass filtered EEG that is not represented by sigma power (window = 5 s, overlap: 2.5 s). n = 4 ( c ); n = 7 ( d , e ). Data is shown as mean ± SEM. * P < 0.05, ** P < 0.01.
Extended Data Fig. 4 Optogenetic suppression of LC.
a , Arch expression in LC was verified by co-staining for TH and GFP. Arrowheads indicate the tip location of the optic fiber (scale bar = 800 µm). b , Close up of a (scale bar = 400 µm). c , Example traces showing norepinephrine level, sigma power, sleep spindles, bandpass LFP in sigma range, and EMG raw data. d , Mean sigma power aligned to onset of NE drop (NREM and IS-REM) or onset of laser stimulation (Arch). e , Time spent in sleep/wake stages during 2-h recording between memory encoding and recall (2-way repeated measures ANOVA with Šídák’s multiple comparison post hoc test). f , Number of laser stimulation (% of total number) in each sleep/wake stage (2-way repeated measures ANOVA with Šídák’s multiple comparison post hoc test). g , Mean NE trace at laser onset during wakefulness. h , NE descent amplitude induced by laser onset during NREM and wakefulness (2-way repeated measures ANOVA with Šídák’s multiple comparison post hoc test, P = 0.0046, NRREM). i , Percentage of laser stimulations during wakefulness resulting in transition to NREM sleep (unpaired t -test). j , Distance moved and ratio between approaches during the recall phase of the novel object recognition (NOR) (two-tailed unpaired t -test, P = 0.049, one-sample t -test P = 0.49, YFP; P = 0.026, Arch). k , Distance moved and object approaches during the acquisition phase of the NOR (unpaired t -test). l , Linear regression between object exploration ratio and time spent in REM sleep. m , Linear regression between object exploration ratio and number of REM bouts/h. n , Linear regression between object exploration ratio and mean theta power during REM sleep. o , Linear regression between object exploration ratio and time spent in NREM sleep. p , Linear regression between object exploration ratio and theta amplitude in response to laser. q , Linear regression between object exploration ratio and delta amplitude in response to laser. n = 9 Arch, 5 YFP. Data is shown as mean ± SEM. * P < 0.05, ** P < 0.01.
Extended Data Fig. 5 Optogenetic activation of LC.
a , Distance moved and ratio between novel and familiar object approaches during recall phase of novel object recognition (ratio: two-tailed unpaired t -test, P = 0.052; one-sample t -test, P = 0.035, YFP; P = 0.64, ChR2). b , Distance moved and object exploration during memory acquisition (right). n = 7 ChR2, 7 YFP. Data is shown as mean ± SEM. * P < 0.05.
Extended Data Fig. 6 The effect of NE reuptake inhibition on EEG and arousability.
a , Representative traces of norepinephrine, raw EEG and EMG before and after administration of the NE reuptake inhibitor, desipramine (des, 10 mg/kg). b , Example EEG and EMG traces from NREM sleep prior to desipramine administration (top), during undefinable phases following desipramine administration (middle) and from defined NREM sleep following administration with desipramine (bottom). c , Sigma power traces aligned to the onset of awakenings (wake EEG/EMG ) following either treatment with saline or desipramine. d , Mean sigma power before and after NREM-wake EEG/EMG transition (2-way repeated measures ANOVA with Šídák’s multiple comparison post hoc test, P = 0.0024, pre; P = 0.031, post). e , Reduction in sigma power amplitude (two-tailed paired t -test, P = 0.0007). f , Distance moved and ratio between novel and familiar object approaches during the recall phase of the novel object recognition (NOR) as well as distance moved and number of object approaches during the acquisition phase (unpaired t -test and one-sample t -test). n = 7 ( c – e ), n = 7 des, 9 sal ( f ). Data is shown as mean ± SEM. ** P < 0.01, *** P < 0.001.
Supplementary information
Supplementary information.
Supplementary Figs. 1–3
Reporting Summary
Source data fig. 1.
Statistical source data
Source Data Fig. 2
Source data fig. 3, source data fig. 4, source data fig. 5, source data extended data fig. 1, source data extended data fig. 2, source data extended data fig. 3, source data extended data fig. 4, source data extended data fig. 5, source data extended data fig. 6, rights and permissions.
Springer Nature or its licensor (e.g. a society or other partner) holds exclusive rights to this article under a publishing agreement with the author(s) or other rightsholder(s); author self-archiving of the accepted manuscript version of this article is solely governed by the terms of such publishing agreement and applicable law.
Reprints and permissions
About this article
Cite this article.
Kjaerby, C., Andersen, M., Hauglund, N. et al. Memory-enhancing properties of sleep depend on the oscillatory amplitude of norepinephrine. Nat Neurosci 25 , 1059–1070 (2022). https://doi.org/10.1038/s41593-022-01102-9
Download citation
Received : 02 August 2021
Accepted : 19 May 2022
Published : 07 July 2022
Issue Date : August 2022
DOI : https://doi.org/10.1038/s41593-022-01102-9
Share this article
Anyone you share the following link with will be able to read this content:
Sorry, a shareable link is not currently available for this article.
Provided by the Springer Nature SharedIt content-sharing initiative
This article is cited by
Treating insomnia with 40 hz light flicker.
- P. Lorenzo Bozzelli
- Li-Huei Tsai
Cell Research (2024)
Microglia regulate sleep through calcium-dependent modulation of norepinephrine transmission
Nature Neuroscience (2024)
Overnight neuronal plasticity and adaptation to emotional distress
- Yesenia Cabrera
- Karin J. Koymans
- Rick Wassing
Nature Reviews Neuroscience (2024)
Cannabidiol Exerts Sedative and Hypnotic Effects in Normal and Insomnia Model Mice Through Activation of 5-HT1A Receptor
- Yu-Meng Liu
- You-Zhi Zhang
Neurochemical Research (2024)
Sleep cycle-dependent vascular dynamics in male mice and the predicted effects on perivascular cerebrospinal fluid flow and solute transport
- Laura Bojarskaite
- Alexandra Vallet
Nature Communications (2023)
Quick links
- Explore articles by subject
- Guide to authors
- Editorial policies
Sign up for the Nature Briefing newsletter — what matters in science, free to your inbox daily.

- News, Analysis & Insights on Nutrition, Supplements, and Health
Bacopa and the brain: Study highlights mood and memory benefits
29-Mar-2024 - Last updated on 29-Mar-2024 at 08:08 GMT
- Email to a friend
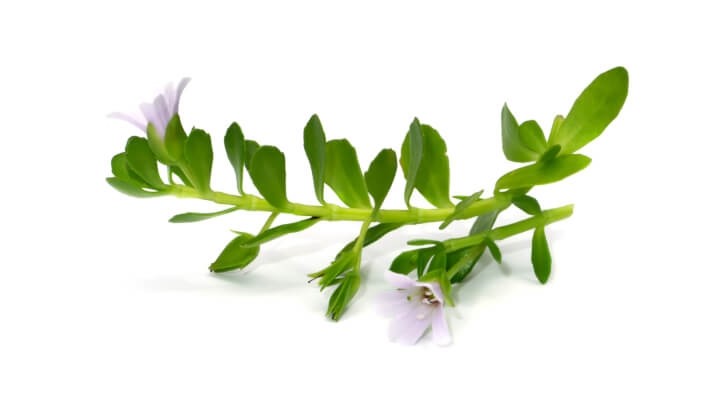
“This is the most comprehensive single study done on Bacopa, and the results of our study demonstrate the potential of Bacopa extract as a safe and effective ingredient to support memory, cognition, stress and sleep quality in healthy adults,” said Aditya Jain, founder and CEO at Samriddh.
The study, published in the Journal of Psychiatry and Cognitive Behavior was conducted by researchers at the BGS Global Institute of Medical Sciences and Leads Clinical Research and Bio Services in Karnataka, India.
Enhancing bacopa bioavailability
Bacopa monnieri , an herb known as Brahmi or water hyssop, has a long history of use in Ayurveda as a nerve-slash-brain tonic. A perennial creeper found in marshy wetlands throughout South and Southeast Asia, its bioactive bacosides are thought to interact with the brain’s dopamine and serotonin systems and promote neuron communication.
“Numerous animal studies have demonstrated cognition-enhancing effects of Bacopa including improved motor learning and memory,” the researchers wrote. “Multiple clinical studies and meta-analysis of clinical studies on cognitive effects of Bacopa have demonstrated its ability to improve cognitive properties including attention.”
The company—which is part of a diversified family business group with interests in consumer goods, steel manufacturing, green energy, real estate, construction and finance—entered the market in 2022 and debuted ingredients B-Lit Bacopa and BeepActive in the U.S. at the most recent Supply Side West trade show in Las Vegas.
Study details
The randomized, double-blind, placebo-controlled trial recruited 80 healthy adults between the ages of 18 and 55 and divided them equally into two groups. One group consumed either 300 mg of B-Lit Bacopa (B. monnieri extract) containing 90 mg of total bacosides and the other a placebo every morning after breakfast for 12 weeks.
Subjects participated in a battery of tests to assess memory, cognitive function, anxiety and sleep quality at different time points. Blood samples were collected to analyze Brain Derived Neurotrophic Factor (BDNF) and cortisol levels, and safety was assessed through the study period.
Key findings included significant improvements in memory, specifically; short-term memory, spatial short-term memory, visuo-spatial memory, working memory and episodic memory, in addition to cognitive improvements related to focus and concentration, alertness, reasoning and mental flexibility compared to placebo. No significant effects were observed for sustained attention and planning tasks.
The researchers also reported key time effects as early as day 14 for cognitive performance and day 28 for memory benefits, as well as an acute effect on concentration three hours after consumption of a single dose. Compared to baseline, reductions in serum cortisol levels were seen at day 56 and day 84 and an increase in BDNF (a major marker of neuroplasticity) on day 84.
“These findings alongside with our technology for enhanced bioavailability and absorption signify a step forward in innovation and research for cognitive health and overall well-being,” Jain said.
Source: Journal of Psychiatry and Cognitive Behaviour doi: 10.29011/2574-7762.000068 “Effect of Bacopa monnieri Extract on Memory and Cognitive Skills in Adult Humans: A Randomized, Double-Blind, Placebo-Controlled Study” Authors: Mohan Muttanahally Eraiah et al.
Related news

- PLT brings new cognitive herb to US
Related products
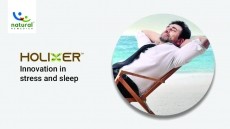
Innovation in Stress and Sleep Management: Holixer
Content provided by Natural Remedies Private Limited | 26-Feb-2024 | White Paper
More than ever, stress is extremely common among adults today.
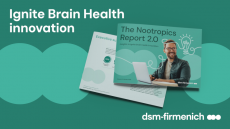
Nootropics Report 2.0: Brain Health Insights
Content provided by dsm-firmenich | 20-Feb-2024 | Insight Guide
The brain health market is constantly growing and evolving, with more consumers looking for innovative ways to support total mind and body wellness.
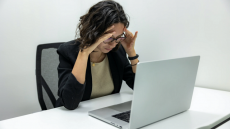
Alleviating Symptoms of Fatigue & Stress with Witholytin®
Content provided by LEHVOSS Nutrition | 08-Feb-2024 | White Paper
Witholytin® is a sustainably produced ashwagandha extract, standardised to a unique profile of withanolides. A new recent study (2023) revealed significant...
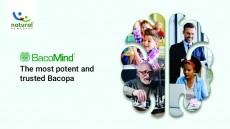
Go Beyond the Ordinary: Cognitive Health-BacoMind
Content provided by Natural Remedies Private Limited | 31-Jan-2024 | White Paper
Experience the potency of BacoMind®, the #1 branded bacopa with 9 key bioactives for unparalleled cognitive support.
- Pycnogenol® for healthy hair and beautiful skin during menopause Horphag Research | Download Technical / White Paper
- Innovation in convenient formats: vegan gummies Cambridge Commodities | Download Product Brochure
- Healthy ageing: a food supplement from Biofarma Group Biofarma Group | Download Technical / White Paper
- Innovation in Stress and Sleep Management: Holixer Natural Remedies Private Limited | Download Technical / White Paper
- Krill oil supports six key areas of healthy aging Aker BioMarine | Download Technical / White Paper
- In Weight Management Go Beyond with Slendacor® PLT Health Solutions | Download Technical / White Paper
Upcoming supplier webinars
- 23 Apr 2024 Tue How does hydrolysate keratin improve Skin, hair, and nails beauty?
- 24 Apr 2024 Wed How to reduce crosslinking and ensure fast dissolution in soft caps
On-demand webinars
- Mitigating safety: Challenges in pet food manufacturing Webinar
- Premium categories: Does the humanization trend still have legs? Webinar
- Sustainable sourcing: Limiting pet industry impact on ecosystems Webinar
- Mining the microbiome: Balance is everything Webinar
- Nutraceutical market trends for 2024: insights by PharmaLinea and IQVIA PharmaLinea Ltd.
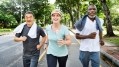
Promotional Features
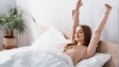
NutraIngredients
- Advertise with us
- Press Releases – Guidelines
- Contact the Editor
- Report a technical problem
- Subscription Benefits
- Why Register
- Whitelist our newsletters
- Editorial Calendar
- Event Calendar

IMAGES
VIDEO
COMMENTS
Most recently, the focus of research in the field has broadened, indicating that sleep benefits memory not only in the neurobehavioral domain, but also in the formation of immunological long-term memories, stimulating the idea that forming long-term memories represents a general function of sleep.
Consolidation during sleep not only strengthens memory traces quantitatively but can also produce qualitative changes in memory representations. An active process of re-organization enables the ...
How sleep shapes what we remember—and forget. Researchers are starting to decode the neural changes during sleep that underlie long-term memory. Our need for sleep is unavoidable. Shortchange yourself a few nights, and you'll incur a debt that not only dulls the senses, but demands longer "recovery" sleep later on ( 1 ).
Sleep. Understanding the complex relationship between sleep and memory consolidation is a major challenge in cognitive neuroscience and psychology. Many studies suggest that sleep triggers off ...
Sleep can benefit memory consolidation. The characterization of brain regions underlying memory consolidation during sleep, as well as their temporal interplay, reflected by specific patterns of brain electric activity, is surfacing. Here, we provide an overview of recent concepts and results on the mechanisms of sleep-related memory consolidation. The latest studies strongly impacting future ...
The beneficial effects of sleep on memory have gained substantial attention in psychology and neuroscience research over the past two decades, resulting in thousands of publications dedicated to ...
Advances in research on memory and sleep can thus shed light on how this processing influences our waking life, which can further inspire the development of novel strategies for decreasing detrimental rumination-like activity during sleep and for promoting beneficial sleep cognition. Menu. Publications A-Z Journal Information
Future research can further probe the types of memory dependent on sleep-related traits and on the neurotransmitters and neuromodulators required. This paper reviews all optogenetic studies that directly test various sleep states, traits, and circuit-level activity profiles for the consolidation of di ... For sleep research in particular, this ...
How Are Memory and Sleep Connected? Sleep and memory Trusted Source National Institutes of Health (NIH) The NIH, a part of the U.S. Department of Health and Human Services, is the nation's medical research agency — making important discoveries that improve health and save lives. View Source share a complex relationship. Getting enough rest helps you process new information Trusted Source ...
Most research shows that sleep plays a critical role in the formation and storage of long-term memories. Different types of memories seem to be processed in different brain regions during certain stages of sleep, especially such phases as rapid eye movement (REM) and slow-wave sleep. Furthermore, sleep has another important function: giving the ...
Sleep benefits the long-term storage of memories [1,2,3,4].However, it is important to note that successful memory relies on at least three distinct processes that are influenced by sleep in ...
Introduction. Several studies primarily in adults have shown that sleep improves procedural memory, i.e. skills and procedures [1], [2] as well as declarative memory [3]. REM and slow-wave sleep (SWS) have been implicated in memory consolidation [3] - [5]. Lack of REM sleep is associated with poor recall of visual location [6].
Nov. 11, 2020 — Sleep is crucial for consolidating our memories, and sleep deprivation has long been known to interfere with learning and memory. Now a new study shows that getting only half a ...
Still, the research suggests there are things we can do to increase the likelihood of a memory being stored permanently. If, like the mice, we pause after an experience, it may help cement the ...
The most promising strategy to initiate an applied sleep-and-memory research program may be to team up with the established basic science sleep-and-memory researchers. Initially, such a program should focus on robust and inexpensive intervention protocols and appliances for healthy participants to establish feasibility for widespread ...
While past studies had linked ripples with memory formation during sleep, the new study, published online March 28 in the journal Science, found that daytime events followed immediately by 5 to 20 sharp wave-ripples are replayed more during sleep and so consolidated into permanent memories. Events followed by very few or no sharp wave-ripples ...
Summary: Researchers uncovered how the brain chooses which daily experiences to convert into long-term memories during sleep, pinpointing "sharp wave-ripples" in the hippocampus as the crucial mechanism.This phenomenon suggests that events followed by sharp wave-ripples are more likely to be consolidated into permanent memories. The research reveals that these ripples occur during idle ...
Over more than a century of research has established the fact that sleep benefits the retention of memory. In this review we aim to comprehensively cover the field of "sleep and memory" research by providing a historical perspective on concepts and a discussion of more recent key findings. Whereas initial theories posed a passive role for sleep enhancing memories by protecting them from ...
The current study aimed to further test the relationship among physical activity, sleep quality, and memory performance in middle-aged and older adults, and to test whether this relationship holds up during the COVID-19 pandemic. ... [Grant 1848972]; and Karen L. Campbell by the Natural Sciences and Engineering Research Council of Canada [Grant ...
Memory dynamics and sleep. Sleep is of fundamental importance to learning. What happens during sleep as the brain consolidates new memories, and how this process can be targeted strategically is a ...
Emerging research suggests that our sleep habits may play a role in shaping how old we feel [6,8]. Sleep is not only crucial for physical well-being but also for mental health. ... This extends previous research showing that undergoing a memory test can lead individuals to feel older, although in older individuals only . Considering that both ...
Ample research has documented a rich microstructural organization of sleep, based on EEG activity patterns. For instance, NREM sleep encompasses both delta-dominated and spindle-rich epochs 1, the ...
Subjects participated in a battery of tests to assess memory, cognitive function, anxiety and sleep quality at different time points. Blood samples were collected to analyze Brain Derived Neurotrophic Factor (BDNF) and cortisol levels, and safety was assessed through the study period.